Sandy Beach Morphodynamics
Edited by
Derek W.T. Jackson Professor
School of Geography and Environmental Sciences
Ulster University, Coleraine
Co. Londonderry, Northern Ireland
United Kingdom
Andrew D. Short Professor
School of Geosciences
University of Sydney
NSW Australia
Elsevier
Radarweg 29, PO Box 211, 1000 AE Amsterdam, Netherlands
The Boulevard, Langford Lane, Kidlington, Oxford OX5 1GB, United Kingdom 50 Hampshire Street, 5th Floor, Cambridge, MA 02139, United States
Copyright © 2020 Elsevier Ltd. All rights reserved.
No part of this publication may be reproduced or transmitted in any form or by any means, electronic or mechanical, including photocopying, recording, or any information storage and retrieval system, without permission in writing from the publisher. Details on how to seek permission, further information about the Publisher’s permissions policies and our arrangements with organizations such as the Copyright Clearance Center and the Copyright Licensing Agency, can be found at our website: www.elsevier.com/ permissions
This book and the individual contributions contained in it are protected under copyright by the Publisher (other than as may be noted herein).
Notices
Knowledge and best practice in this field are constantly changing. As new research and experience broaden our understanding, changes in research methods, professional practices, or medical treatment may become necessary.
Practitioners and researchers must always rely on their own experience and knowledge in evaluating and using any information, methods, compounds, or experiments described herein. In using such information or methods they should be mindful of their own safety and the safety of others, including parties for whom they have a professional responsibility.
To the fullest extent of the law, neither the Publisher nor the authors, contributors, or editors, assume any liability for any injury and/or damage to persons or property as a matter of products liability, negligence or otherwise, or from any use or operation of any methods, products, instructions, or ideas contained in the material herein.
Library of Congress Cataloging-in-Publication Data
A catalog record for this book is available from the Library of Congress
British Library Cataloguing-in-Publication Data
A catalogue record for this book is available from the British Library
ISBN: 978-0-08-102927-5
For information on all Elsevier publications visit our website at https://www.elsevier.com/books-and-journals
Publisher: Candice Janco
Acquisitions Editor: Louisa Munro
Editorial Project Manager: Naomi Robertson
Production Project Manager: Omer Mukthar
Designer: Mark Rogers
Front cover image: Downhill beach looking toward Magilligan Point, Northern Ireland, UK
Back Cover image: Back Banks Beach, on Robbins Island, Bass Strait, Tasmania
Typeset by Thomson Digital
Contributors
Stefan Aarninkhof Delft University of Technology, Delft, The Netherlands
Andrew D. Ashton Department of Geology and Geophysics, Woods Hole Oceanographic Institution, Woods Hole, MA, United States
Tom E. Baldock School of Civil Engineering, University of Queensland, Brisbane, QLD, Australia
Tom Beuzen Department of Statistics, University of British Columbia, Vancouver, Canada
Judith Bosboom Department of Hydraulic Engineering, Delft University of Technology, Delft, The Netherlands
Karin R. Bryan School of Science & Environmental Research Institute, University of Waikato, Hamilton, New Zealand
Helene Burningham Department of Geography, University College London, London, United Kingdom
Bruno Castelle CNRS; UMR EPOC, University of Bordeaux, Pessac, France
Giovanni Coco School of Environment, University of Auckland, Auckland, New Zealand
Ana Vila-Concejo Geocoastal Research Group, School of Geosciences F09, The University of Sydney, Sydney, NSW, Australia
Ana P. da Silva Griffith Centre for Coastal Management, Griffith University, Gold Coast, QLD, Australia
Sierd de Vries Department of Hydraulic Engineering, Delft University of Technology, Delft, The Netherlands
Kees den Heijer Delft University of Technology, Delft; Data2day, Pijnacker, The Netherlands
Miriam Fernandez-Nunez Department of Geography, University College London, London, United Kingdom
Óscar Ferreira Centre for Marine and Environmental Research, Faculty of Sciences and Technology, University of Algarve, Faro, Portugal
Burghard Flemming Senckenberg Institute, Suedstrand, Wilhelmshaven, Germany
Shari L. Gallop School of Science & Environmental Research Institute, University of Waikato, Hamilton & Tauranga, New Zealand
Matthijs Gawehn Deltares; Delft University of Technology, Delft, The Netherlands
Lluís Gómez-Pujol Earth Sciences Research Group, Department of Biology, University of the Balearic Islands, Mallorca, Spain
Ian D. Goodwin Marine Climate Research Group, Department of Earth and Environmental Sciences, Macquarie University, NSW, Australia
Emilia Guisado-Pintado Physical Geography and Regional Geographic Analysis Department, Universidad de Sevilla, Seville, Spain
Mitchell Harley Water Research Laboratory, School of Civil and Environmental Engineering, UNSW Sydney, Sydney, NSW, Australia
Chris Houser School of the Environment, University of Windsor, Windsor, ON, Canada
Michael G. Hughes Science Division, NSW Department of Planning Industry and Environment, Sydney, NSW; School of Earth Atmosphere and Life Sciences, University of Wollongong, North Wollongong, NSW, Australia
Christopher J. Hein Department of Physical Sciences, Virginia Institute of Marine Science, William & Mary, Gloucester Point, VA, United States
Derek W.T. Jackson School of Geography and Environmental Sciences, Ulster University, Coleraine, Northern Ireland, United Kingdom
Antonio H.F. Klein Special Coordination of Oceanography, Center for Physical and Mathematical Sciences, Federal University of Santa Catarina (UFSC), University Campus Trindade, Florianópolis, Brazil
Alejandro López-Ruiz Department of Aerospace Engineering and Fluid Mechanics, University of Seville, Seville, Spain
John L. Largier Coastal & Marine Sciences Institute, University of California Davis, Bodega Bay; Department of Environmental Science & Policy, University of California Davis, Davis, CA, United States
Mark Lee HR Wallingford Ltd. Oxfordshire, United Kingdom
Summer Locknick School of the Environment, University of Windsor, Windsor, ON, Canada
Miguel Á. Losada Andalusian Institute for Earth System Research, University of Granada, Granada, Spain
Carlos Loureiro Biological and Environmental Sciences, University of Stirling, Stirling, United Kingdom; Geological Sciences, University of KwaZulu-Natal, Durban, South Africa
Arjen Luijendijk Deltares; Delft University of Technology, Delft, The Netherlands
Fernando J. Méndez Grupo de Ingeniería Geomática y Oceanográfica. GeoOcean. Dept. CYTAMA. E.T.S.I.C.C.P. Universidad de Cantabria, Santander, Cantabria, Spain
Thomas Mortlock Marine Climate Research Group, Department of Earth and Environmental Sciences, Macquarie University, NSW, Australia
Brad Murray Division of Earth and Ocean Sciences, Nicholas School of the Environment; Center for Nonlinear and Complex Systems, Duke University, Durham, NC, United States
Alejandro Orfila IMEDEA (CSIC-UIB), Mediterranean Institute for Advanced Studies, Mallorca, Spain
Miguel Ortega-Sánchez Andalusian Institute for Earth System Research, University of Granada, Granada, Spain
Luci Pereira Universidade Federal do Pará, Pará, Brazil
Hannah E. Power School of Environmental and Life Sciences, University of Newcastle, Callaghan, NSW, Australia
Marta Ribó Marine Climate Research Group, Department of Earth and Environmental Sciences, Macquarie University, NSW, Australia
Ana Rueda Surf and Surge Research Group, GeoOcean, University of Cantabria, Santander, Spain
Amaia Ruiz de Alegría-Arzaburu Institute of Oceanographic Research, Autonomous University of Baja California, Ensenada, Baja California, México
Nadia Senechal University of Bordeaux, OASU-UMR EPOC, Pessac, France
Andrew D. Short School of Geosciences, The University of Sydney, Sydney, NSW, Australia
Kristen Splinter Water Research Laboratory, School of Civil and Environmental Engineering, UNSW Sydney, Sydney, NSW, Australia
Rui Taborda Faculty of Sciences, University of Lisbon, Instituto Dom Luiz, Campo Grande, Lisbon, Portugal
Bruce Thom University of Sydney, School of Geosciences, Sydney, NSW, Australia
Anne Ton Delft University of Technology, Delft, The Netherlands
Sarah Trimble Department of Geography, Texas A&M University, College Station, TX, United States
Wellington Trindade Universidade Federal do Pará, Pará, Brazil
Guilherme Vieira da Silva Griffith Centre for Coastal Management, Griffith University, Gold Coast, QLD, Australia
Sander Vos Delft University of Technology, Delft; Baars-CIPRO, Lijnden, Holland, The Netherlands
Ian J. Walker School of Geographical Sciences and Urban Planning, School of Earth and Space Exploration, Arizona State University, Tempe, AZ, United States
Meagan Wengrove Oregon State University, Corvallis, OR, United States
Phil Wernette School of the Environment, University of Windsor, Windsor, ON, Canada
Gundula Winter Unit of Marine and Coastal Systems, Deltares, Delft, The Netherlands
Introduction to beach morphodynamics
Derek W.T. Jacksona, Andrew D. Shortb
aSchool of Geography and Environmental Sciences, Ulster University, Coleraine, Northern Ireland, United Kingdom; bSchool of Geosciences, The University of Sydney, Sydney, NSW, Australia
Chapter outline
1.1 Introduction 1
1.1.1 Chapter content 4
1.1.2 Emerging developments in the study of sandy beaches 8 References 11
1.1 Introduction
The term ‘morphodynamics’ was introduced into the coastal literature by Wright and Thom (1977) who presented it as a way to better understand the linkages and feedbacks between processes and morphological responses in the coastal zone. As the title of their paper indicates, they saw it applying to all coastal landforms. Its application to beaches quickly followed, with Wright et al. (1979), to wave–beach–dune interactions with Short and Hesp (1982) and to Holocene coastal evolution (Short and Fotheringham, 1986). Since then the term has become embedded in the coastal literature though not always with the correct intent.
The origins of the morphodynamic approach, however, predate the 1977 paper and can be traced back to the adventurous group of coastal scientists at Louisiana State University’s Coastal Studies Institute (CSI), who roamed the world’s coasts and in the process, developed a holistic approach looking at the interaction between form (morphology) and process (dynamics) in their investigation of coastal systems (Short and Jackson, 2013). This approach was embedded in their work on the world’s delta (e.g. Wright and Coleman, 1971) and then beaches, particularly with their pioneering ‘SALIS’ (sea–air–land interaction) experiments (Sonu, 1972, 1973; Sonu et al., 1973). Not surprisingly Thom, Wright and Short, who were all CSI graduates, took the approach to Australia, where they called it ‘morphodynamics’.
At the core of landform morphodynamics is both the interrelationship between the bed response (morphology) to processes (dynamics) and the ensuring positive and negative feedbacks between the two as they work towards an equilibrium relationship, which is rarely achieved, hence driving change (Fig. 1.1). It involves both the spatial and temporal domains at scales from the instantaneous (e.g. suspended sediment) through to millennium (e.g. Quaternary coastal evolution) (Fig. 1.2; Cowell and
Sandy Beach Morphodynamics. http://dx.doi.org/10.1016/B978-0-08-102927-5.00001-1
Copyright
Figure 1.1 The morphodynamic relationships between boundary conditions (topography), inputs, interactions (central boxes) and resulting surface morphology (topography), and underlying stratigraphy in the coastal environment. Reproduced from Cowell, P.J., Thom, B.G., 1994. Morphodynamics of coastal evolution. In: Carter, R.W.G., Woodroffe, C.D. (Eds.), Coastal Evolution: Late Quaternary Shoreline Morphodynamics. Cambridge University Press, Cambridge, pp. 33–86.
Thom 1994), and as such it invokes the full spectrum of coastal morphology from ripples through to barriers, deltas and headlands, at all time-scales. It is, however, more than this time–space domain, as it must also embed the ongoing interactions and feedback between the two mutually antagonistic parameters: on the one hand, sediment and geology are held firm by gravity and structure; on the other, waves, wind and currents attempt to overcome gravity and mobilise, transport, sort and deposit the material. In the process they arrange the sediment/geology it into the full spectrum of coastal landforms, which in doing so impede the processes as the landform stabilises, temporarily or permanently. It is this ongoing interaction and its outcomes, which are at the core of morphodynamics.
Sandy beaches provide one of the most interesting and profitable environments in which to use the morphodynamics approach. They are one of the most dynamic environments on earth and one of the most responsive, much visible with the naked eye. As shoaling waves approach the shore, their wave orbital motions mobilise sediment and imprint a predictable sequence of ripples on the seabed, followed by the turbulence of wave breaking and a surf zone with gravity through infragravity waves and on, off and along-shore currents all combined with tides, to arrange the sand into
Figure 1.2 The relationship between the scale of coastal sedimentary features and their temporal variability, together with the four major time–space paradigms used in the study of coasts.
Reproduced from Cowell, P.J., Thom, B.G., 1994. Morphodynamics of coastal evolution. In: Carter, R.W.G., Woodroffe, C.D. (Eds.), Coastal Evolution: Late Quaternary Shoreline Morphodynamics. Cambridge University Press, Cambridge, pp. 33–86.
a predictable sequence of beach states and types (Short 2006; see Chapters 13, 16). As most beach studies focus on a component of the beach system, usually over a limited time frame, it is essential that they adopt the appropriate length and time scales to both observe the processes and the bed responses and observe the feedback between the two, as indicated in Fig. 1.2.
This book is designed to provide an up-to-date overview of the state of sandy beach morphodynamic research and publication as of 2020. Over 60 leading coastal scientists have combined to write the 29 chapters, with the chapter authors focusing on their area of expertise. The following section briefly reviews the content of each chapter followed by a final section on new and emerging developments in the study of sandy beaches.
1.1.1 Chapter content
This reference book steps through various aspects of sandy beach morphodynamics, ranging from the type and nature of sandy material that constitutes beaches to the
management of such systems. Chapter 2, Beach sand and its origins, takes us through some of the origins of sandy material found on beaches, exploring sources of sediment and formational processes and ultimately how it becomes arranged into the main beach framework. Beginning in deeper waters, Chapter 3, Wave climates: Deep water to the shoaling zone, outlines what is one of the chief forcing agents in beach systems and explores the wave climate off coastlines, taking a longer-term view of wave climate regimes and their implications for beach morphodynamics. As waves approach shallower depths at the coast, they begin to be influenced by the underlying seabed type and its bathymetry, and Chapter 4, Wave behaviour outside the surf zone, looks at how waves initially form and then in coastal waters begin to shoal as they interact with the shoaling seabed and begin to transfer some of their energy before the surf zone is reached. Key processes such as wave refraction, energy dissipation, white-capping, bottom-friction, diffraction and linear wave theory are examined. Beach morphodynamics can also be strongly affected by tidal elevation differences, repositioning wave shoaling and breaking to varying degrees depending on the tide range and beach slope at individual sites. Chapter 5, Tidal modulation, discusses the impact of meso- and mega-tidal ranges at a selection of beach locations from around the world. The chapter looks at how tidal modulation varies according to the tidal cycles, seasonal variation, offshore wind patterns, major weather events and astronomical forces, exacting a significant influence on morphodynamics at tidally influenced beaches. Chapter 6, Breaking waves, looks at what happens as waves progress into shallower conditions eventually breaking Entering the surf zone, initial wave breaking processes ensue as when the depth becomes too shallow for stable waveforms to maintain themselves. This chapter demonstrates the wide distribution of individual wave heights found across the surf zone and how these can be modelled and observed.
Chapter 7, The surf zone, presents the hydrodynamics of the surf zone and how it affects sediment transport and beach morphodynamics. The chapter shows the influence of breaking waves and their role in altering water levels and surf zone processes, which ultimately help drive surf zone circulation patterns. The various types of infragravity waves are also explored along with key types of currents found in the surf zone. Finally, this chapter also describes how geological control can modify surf zone processes. Moving to the very shallow part of the coastal zone, Chapter 8, The swash zone, describes the morphological features and key processes found in the swash zone. Located at the landward edge of the inundated part of the beach system, incoming wave bores help force oscillatory motions of the shoreline. The chapter examines the beach face slope, beach berms, beach steps and beach scarps as well as sediment sorting across the beach face profile. Recent developments in swash flow kinematics/ dynamics and sediment transport mechanics are discussed in relation to an improved understanding of swash zone morphodynamics. Current research directions and outstanding issues still to be resolved in the swash zone are also discussed.
The morphodynamics of sandy beaches are effectively directed by how sediment is transported and deposited. This involves processes involved in subaqueous and subaerial sediment transport; however, quantifying these has proven to be a difficult challenge. Chapter 9, Marine sediment transport, provides an overview of the most important concepts currently used to describe sediment transport processes, examining
sediment transport as a function of various hydrodynamic processes such as waves, currents and turbulent forces. The chapter outlines the most important modes of sediment transport in both the cross-shore and longshore and summarises some of the methods used to measure transport. Sandy beaches are also subjected subaerial forces through the action of wind, a highly capable geological agent responsible for transporting sand away from wave action and depositing it in the form of back-beach dunes. Chapter 10, Aeolian (wind-blown) sand transport on beaches, reviews our current understanding of the measurement and modelling of windblown sand transport over beaches. The fundamentals of boundary layer airflow dynamics and aeolian sand transport are explained, and key transport models are classified. Key environmental controls that affect aeolian processes are outlined, and their influence on transport mechanics and modelling are discussed. The chapter focuses primarily on empirical field and wind tunnel research findings and identifies key advances and current challenges for aeolian research in coastal beach settings.
Chapter 11, Rip currents, examines a topical phenomenon found on many sandy beach systems, which is particularly relevant to our understanding of beach hazards. The chapter focuses largely on the development and evolution of rip currents as part of the natural beach and nearshore system and examines sediment transport processes associated with the evolution of the nearshore in the presence of rip currents. Here, rips are described as a ‘feedback’ to the local nearshore morphology and therefore an essential part of beach and nearshore recovery following storm wave events.
Chapter 12, From cusps to capes: Self-organised shoreline shapes, addresses the imprinted patterns of fluid flow on the beach landscape and the fluid–sediment system behaviour, applying the concept of self-organisation as an explanation of shortto longer-term dynamics and the resulting beach landforms observed. How and why rhythmic patterns in the surf zone develop has been an enduring conundrum for coastal scientists. Chapter 13, Rhythmic patterns in the surf zone, outlines the various sandbar patterns that develop in this highly energetic zone such as multiple sandbars, crescentic sandbars and transverse sandbars. The chapter describes how different settings and wave conditions can lead to different morphodynamic behaviour and bar patterns. A number of theories are presented that have attempted to explain the formation of these rhythmic patterns but the chapter focuses on new developments in this field over the last decades that are based on ‘self-organization’ theory to explain pattern emergence in the surf zone.
On occasions, sandy beaches can become mixed with gravel-sized sediment across and along the beach profile. These types of beaches can be found in a wide range of tidal and wave environments and particularly in higher latitude and collision coasts where coarse material is more common, but despite their complexity they have received relatively less attention than purely sandy beaches. Chapter 14, Mixed sand and gravel beaches, explores the main physical processes that control their morphodynamics and looks at their various unique qualities as well as how they behave to wave forcing.
Sandy beaches are deposited by waves within a tidal environment and as such are influenced by both. In particular, low wave energy settings such as in bays and estuaries beaches they can be ubiquitous features. Chapter 15, Sandy beaches in estuaries
and bays, outlines some of the unique qualities of these beaches as well as their management. The chapter examines the role played by local geology and sediment availability and the importance of energy gradients with particular reference to the shape and size of the ocean (estuarine) entrances, the bathymetry of the ocean entrance itself and its influence on waves, and finally their exposure to tide and fluvial currents. On open coasts higher waves can dominate beach behaviour. However, as waves decrease and tide range increases, the two interplay to provide a spectrum of beach types and states. Chapter 16, Wave-dominated, tide-modified and tide-dominated continuum, examines the wide range in wave-tide combinations, as expressed by the relative tide range (TR/Hb), and outlines attributes of wave-dominated (WD), tide-modified (TM) and tide-dominated (TD) systems. This chapter presents the spectrum of beach types and states and categorises them within a classification system and also examines some of the spatial controls in their placement and distribution.
As waves are a primary carrier of energy in sandy beach systems, knowing their attributes is vitally important in our understanding of coastal processes. However, data on wind and wave characteristics are often only available in the open ocean, whereas in shallow water, where they interact most with the seabed through processes such as refraction, diffraction and wave breaking, in situ wave measurements are often absent. Wave modelling therefore has helped fill these data gaps and provided much needed information on spatial and temporal behaviour of wave behaviour in shallow water nearshore environments. Chapter 17, Shallow water wave modelling in the nearshore (SWAN), takes us through the origins of nearshore modelling and how the shallow water wave model SWAN has now become ubiquitous in many sandy beach studies in recent years. In recognition of waves and currents interacting at different spatial and temporal scales to form a beach profile configuration, a commonly used model is the ‘wave-dominated’ beach model that defines six main beach states based on the use of the dimensionless fall velocity (Ω). Chapter 18, The reflective-dissipative continuum, describes the model which incorporates three-dimensional beach schema results in distinctive beach ‘states’. The chapter outlines the importance of forcing processes (wave height, period and sediment size) and the resulting physical beach profile that arises and frames the continuum morphodynamic character of wave-dominated beach systems, namely dissipative, intermediate and reflective.
Most sandy beach coastlines are not static, and for a longer term view of system dynamics they can be examined using shoreline change analysis, whereby shifts in the position of the shoreline are monitored over different time frames using a number of data sources. Chapter 19, Shoreline change analysis, explores the various workflows and procedures involved in shoreline change analysis and how it has evolved over recent decades, examining change at multiple temporal and spatial scales. The chapter reviews the development of the concept and the various tools associated with the analysis using a worked example from the UK. Chapter 20, Seasonal imprint on beach morphodynamics, reviews the effects of seasonality on sandy beach morphodynamics. Seasonality can be significant for beach systems and often shows distinctive and observable intra-annual variability. The chapter highlights why this is important for beach management and then moves onto the notion of seasonality genesis, evidenced by wave-driven, aeolian-driven and rain-driven
seasonality imprints. Other aspects covered include data collection and data-driven models (Empirical Orthogonal Function (EOF) analysis, neural network, equilibrium model) as well as morphological and process-based approaches in understanding seasonality.Chapter 21, Long-term shoreline change: Processes and preservation of environmental signals, then continues the longer-term theme of beach morphodynamics and examines the factors that control the longer-term evolution of beach and their associated barrier systems as well as the preservation potential of paleo-environmental archives. It presents global examples of these longer-term paleo-signatures and the challenges associated with their analysis. Chapter 22, Extreme events: Impact and recovery, outlines further longer-term beach behaviour, this time considering the higher-energy, more extreme storm events. Operating on time scales from days to years up to decades, beach changes may result from the erosion-recovery (im)balance forced by storms and recovery conditions. Thanks to both long-term and rapid-response coastal monitoring programs we have seen advances over the last decade in our understanding (and predictive ability) of storm-driven erosion and subsequent multiday to multiannual recovery. The chapter provides a broad overview of the definitions and processes controlling storm-driven beach and dune erosion and subsequent recovery. This is examined further through the use of two case studies and storm clustering analysis and event response/recovery from beach sites in Europe and Australia.
Chapter 23, Headland bypassing and overpassing: Form, processes and applications, covers the present status of headland bypassing (HB) and overpassing (HO) processes, essential in many headland-bound coastal sediment budgets, where they connect coastal cells and support the continuity of the sediment transport around or over headlands. The chapter gives an initial overall definition and outlines the evolution of the research area. Sediment characteristics and availability, geomorphological constrains and driving forces are described, along with conceptual models and methods used to investigate bypassing and overpassing. The chapter concludes with case examples incorporating the main concepts highlighted and future perspectives on the topic.
Longshore and/or cross-shore sediment transport driven by seasonal or periodic changes in wave direction and/or gradients in wave energy can result in what is known as ‘beach rotation’. Chapter 24, Mechanisms and time scales of beach rotation, outlines the processes involved, including the complex mechanisms, drivers and time scales of beach rotation that arise from the nonlinear interactions of cross-shore and alongshore hydrodynamic forcing, sediment transport and morphological changes. The chapter also discusses the impacts from rising sea levels and changes in wave climate and finally concludes with some of the challenges associated with process-based modelling of embayed beach morphodynamics.
Chapter 25, Coastal sediment compartments, wave climate and centennial-scale sediment budget, explores sediment transport and exchange between compartments along coastlines, incorporating wave climate, planform embaymentisation and headlands within the context of a time-varying sediment budget. Using a case study from southeastern Australia, centennial-scale sediment budgets are assessed to reveal past, present and future coastal evolution. The latest approaches to understanding coastal
stability and sediment budgets in response to changing sea levels and wave climate are discussed using a range of modelling methodologies.
Surprisingly, despite its heavily populated and developed nature in many areas, until recently coastal zones had no reliable global-scale assessment of historical shoreline change trends. Chapter 27, Global beach database, discusses a new, cloudbased image analysis technology developed to determine shoreline dynamics over the three past decades. Using freely available optical satellite images captured since 1984 and combined with sophisticated image interrogation and analysis methods, the prevalence of sandy beaches and their rates of shoreline change are assessed at global scale using pixel-based supervised classification. A global data set of shoreline change rates spanning 1984–2016 has been generated enabling more quantified rates of retreat to be analysed. The methods developed provide a unique opportunity to retrieve historic information on shoreline dynamics at a decadal scale for any beach in the world.
Chapter 28, Machine learning and coastal processes, describes the use of specialised computing approaches to understanding complex environmental systems such as beach morphodynamics. Machine learning involves the use of algorithms for automatically finding and relating patterns in data and can involve algorithms from simple linear regression to complex multilayer neural networks. This chapter covers the basic concepts of machine learning including neural networks, support vector machines, Bayesian networks, decision trees and the k-nearest Neighbours algorithm and leads onto examples applied to coastal processes. It also includes the steps required to develop machine learning algorithms with a worked case study and concludes with a brief discussion on the future of machine learning in coastal processes research.
Finally, Chapter 29, Future challenges in beach management as contested spaces, deals with the (contested) management of sandy beach systems. With a diverse list of uses of sandy beaches inevitably this attracts contention. Built structures on or adjacent to active sandy beaches can impede or disrupt sediment movements that can ultimately impact on public use of the beach. Increased coastal populations and resulting development pressures, combined with climate change, will exacerbate predicted impacts of extreme storm events and therefore require the management of beaches to access a range of technical advice from geomorphology and coastal engineering to socio-economic and legal disciplines to help address the challenges of beach use, resilience and sustainability.
1.1.2 Emerging developments in the study of sandy beaches
Developments in technology have allowed rapid advances in how scientists record, measure and analyse environmental data in natural sciences. Sandy beach systems are subject to physical forcing through a whole suite of environmental variables operating over multiple space and time scales. Until recently, many of these variables were not being adequately captured at sufficient spatial or temporal scales to produce meaningful data sets with which to properly understand (and manage) these systems. Technological progress has however helped bridge these gaps in data collection and has driven significant recent progress in our understanding of sandy beach systems.
As a result, both remote and in situ observational technologies capturing processes and form have led to substantially larger and more accurate data sets, which have significantly improved the ability to map and monitor both beach change and forcing processes, which in turn have improved modelling performance (Fringer et al., 2019).
At the larger scale, particularly when capturing far-field marine data, in recent decades coastal ocean observation systems have been established at national or regional level in the United States, Australia, Europe and elsewhere. Initial coastal monitoring systems were aimed specifically at operational applications, climate studies and general environmental assessment with little or no integrated approach adopted. Integrated coastal ocean observing systems have recently been designed and developed to fit multiple purposes. In the United States, the Integrated Ocean Observing System (IOOS) is a national observing setup to monitor its coastal shelf sea waters, under the control of several regions (Corredor, 2018). In Australia, the Integrated Marine Observing System (IMOS, Hill et al., 2009; Turner et al. 2011), although similar to the United States’ system, was designed with a more research agenda in mind, targeting operational forecasting and management of its natural marine resources. Both IOOS and IMOS have modern technologies as their platform, helping to feed into the Global Ocean Observing System (GOOS) coastal and biological observations (Moltmann et al., 2019). The institutional membership of the European Regional Operational Oceanography Systems (ROOS) consists of the UK’s Meteorological Office, the National Oceanography Centre (NOC) and the Natural Environment Research Council (NERC); Spain’s Puertos del Estado, the Spanish Institute of Oceanography (IEO); The Portuguese Hydrographic Institute (IH); France’s Mercator Ocean, Ireland’s Marine Institute and the Institut français de recherche pour l’exploitation de la mer (Ifremer) in France. These all feed into the EuroGOOS which adopts an integrated use of multiple technologies, integrating these regional observational networks with online delivery, and an open and free access functionality.
Remote-sensing (space to earth) platforms of global down to regional- and even local-scale features and environmental conditions have allowed us to progressively examine the coastline and specifically beach changes with rapidly increasing resolution and frequency (e.g. Splinter et al. 2018). Sensor development on these platforms incorporating optical, spectral and multispectral technology with enhanced image resolution, now allows multiple types of information to be extracted regularly to help build up a temporal sequence of change. Recently, this has enabled us to examine global patterns of beach/shoreline changes (Luijendijk et al., 2018; Chapter 26).
Nearshore bathymetry continues to be the ‘Achilles heel’ in coastal investigations, and even though ship-mounted, multibeam techniques continue to provide nearshore bathymetric mapping data, very shallow (0–10 m) nearshore depth monitoring is time-consuming and not favourably disposed to low-cost, repeat surveying. As such, recent efforts have focused on using satellite imagery for bathymetric extraction, although this can have limitations due to cloud cover and water clarity issues (Yunus et al., 2019; Pacheco et al., 2015). Recently, NASA’s Ice, Cloud and land Elevation Satellite 2 (ICESat-2) has been providing shallow water bathymetric information in coastal waters (if turbidity permits) and adds further options to this important interface area of coastal beaches.
The ability to remotely sense individual beach systems with the use of video and still images obliquely targeting nearshore zones (Stringari and Power, 2019; Bergsma et al., 2019), beach zones and shoreline positions has seen steady progress in recent years with developments in sensor quality and affordability along with more turn-key analytical tools being available. The use of off the shelf, relatively inexpensive Unmanned Autonomous Vehicles (AUV) drone platforms, has produced a plethora of research monitoring efforts aimed at capturing repeat vertical imagery of beach and dune sites to enable short- and long-term monitoring (e.g. Harley et al., 2015). Structure from Motion (SfM) techniques from supplied drone software now enable three-dimensional surface models to be generated for difference mapping of landform changes (e.g. Guisado-Pintado et al. 2018). Airborne and ground-based laser mapping systems continue to evolve, producing invaluable topographic detail of beach and nearshore changes in surface elevations (e.g. Harley et al., 2017). Airborne LiDAR remains expensive though and out of the reach of lower budget monitoring programs, with the latter drone techniques now replicating some of the topographic acquisition needs of researchers. Ground-based terrestrial laser scanning (TLS) instrumentation is also still largely expensive for most research teams but does produce extremely detailed relief information for many purposes such as beach topographical changes where SfM techniques may struggle photogrammetrically to produce surface models where features are not well defined on flatter beaches. Intertidal areas can also be mapped and monitored using standard marine navigation radar (X-band frequency) within a temporal domain (Bell et al., 2016). This can highlight important behaviour of waterline patterns and inundation with clear advantages over standard optical cameras as it can be used in low light conditions.
A good example of a developing citizen science initiative involving members of the public contributing to beach monitoring is CoastSnap (Harley et al. 2019). This involves the use of members of the public taking and uploading mobile phone images from fixed platforms overlooking sandy beach systems, helping build a chronology of images that are uploaded, stored and subsequently analysed by experts. This represents an inexpensive monitoring technique to also extract shoreline change information over time. Pre- and post-storm impacts (and recovery) can also be gleaned from these approaches (Harley et al. 2017)
Advances being made in field technology to monitor both processes and the nature of (seabed) and changes in its morphology ultimately inform us how wave and current processes interact over these changing morphologies. The seabed and its subsurface are being mapped in increasing detail using sonar and swath mapping (e.g. Linklater et al., 2019), and the beach and dune surfaces and subsurface are being mapped with LiDAR and Ground Penetrating Radar (GPR) (e.g. Oliver et al., 2017). The processes driving change and sediment transport are being monitored with an increasing compact and a sophisticated range of sensors (e.g. Christensen et al. 2018; McCarroll et al., 2018) as outlined in Chapters 9, 10 and 27. The integration of these techniques enables the coast and its beach systems to be monitored and modelled at scales from instantaneous (Chapters 6, 7 and 8), to events (Chapter 22), to decadal (Chapters 19, 23 and 25) to millennium (e.g. Oliver et al., 2017).
Computer modelling will likely take a much more prominent position in sandy beach morphodynamics in the future, particularly with the advances in computing power now available. Wave climate hindcasting, monitoring and modelling (Chapter 3) coupled with SWAN modelling (Chapter 17) and good-quality bathymetry data sets being generated now allow more accurate modelling of nearshore and breaking wave height (Chapters 4 and 6) and, through this, better prediction of beach response (Reeve et al., 2016, 2019; Simmons et al., 2019). Measurement of significant wave height offshore is important for a whole host of reasons ranging from wave model validation to understanding wave energy delivery to beach systems and novel techniques using microwave remote-sensing methods such as (marine) X-band radar are showing promise (Salcedo-Sanz et al., 2015). Marine (ship-mounted) radar is normally only used for navigation and not oceanographic research but with the correct post-processing procedures it may prove to be a useful future tool in gleaning significant wave heights.
Shoreline change models for the prediction of future coastline configuration also continue to show great potential (Davidson et al. 2013; Splinter et al., 2014) but more validating field studies are still required. Backed up by in situ measurements and remote-sensing advances the validation tools are there for new modellings efforts. Using approaches such as machine learning (Chapter 28) excites new avenues for sandy beach morphodynamics, especially when parallel processing is now much more available either through local computing clusters or via cloud computing.
References
Bell, P.S., Bird, C.O., Plater, A.J., 2016. A temporal waterline approach to mapping intertidal areas using X-band marine radar. Coast. Eng. 107, 84–101.
Bergsma, E.W.J., Conley, D.C., Davidson, M.A., O’Hare, T.J., Almar, R., 2019. Storm event to seasonal evolution of nearshore bathymetry derived from shore-based video imagery. Remote Sens. 11 (519.)
Christensen, D.F., Brinkkemper, J., Ruessink, G., Asgaar, T., 2018. Field observations of turbulence in the intertidal and shallow subtidal zones. Cont. Shelf Res. 170, 21–32
Cowell, P.J., Thom, B.G., 1994. Morphodynamics of coastal evolution. In: Carter, R.W.G., Woodroffe, C.D. (Eds.), Coastal Evolution: Late Quaternary Shoreline Morphodynamics. Cambridge University Press, Cambridge, pp. 33–86
Corredor, J.E., 2018. Coastal Ocean Observing - Platforms, Sensors and Systems. Springer International Publishing, Berlin
Davidson, M.A., Splinter, K.D., Turner, I.L., 2013. A simple equilibrium model for predicting shoreline change. Coast. Eng. 73, 191–202
Fringer, O.B., Dawson, C.N., He, R., Ralston, D.K., Zhang, Y.J., 2019. The future of coastal and estuarine modeling: findings from a workshop. Ocean Model. 143, 101458
Guisado-Pintado, E., Jackson, D.W.T., Rogers, D., 2018. 3D mapping efficacy of a drone and terrestrial laser scanner over a temperate beach-dune zone. Geomorphology 328, 157–172
Harley, M.D., Turner, I.L., Short, A.D., Bracs, M.A., Phillips, M.S., Simmons, J.A., Splinter, K., 2015. Four decades of coastal monitoring Narrabeen-Collaroy Beach: the past, present and future of this unique dataset. Australasian Coast & Ports Conference, Auckland, 6 pp.
Sandy Beach Morphodynamics
Harley, M.D., Turner, I.L., Kinsela, M.A., Middleton, J.H., Mumford, P.J., Splinter, K.D., Phillips, M.S., Simmons, J.A., Hanslow, D.J., Short, A.D., 2017. Extreme coastal erosion enhanced by anomalous extratropical storm wave direction. Sci. Reports 7, 6033
Harley, M.D., Kinsela, M.A., Sanchez-Garcia, E., Vos, K., 2019. Shoreline change mapping using crowd-sourced smartphone images. Coast. Eng. 150, 175–189
Hill, K.L., Moltmann, T., Proctor, R., Allen, S., 2009. Australia’s integrated marine observing system. Meteorol. Technol. Int. 1, 114–120
Linklater, M., Ingleton, T.C., Kinsela, M.A., Morris, B.D., Allen, K.M., Sutherland, M.D., Hanslow, D.J., 2019. Techniques for classifying seabed morphology and composition on a subtropical-temperate continental shelf. Geosciences 9, 141
Luijendijk, A., Hagenaars, G., Ranasinghe, R., et al., 2018. The State of the World’s Beaches. Sci Rep 8 6641, doi:10.1038/s41598-018-24630-6
McCarroll, R.J., Masselink, G., Valiente, N.G., Scott, T., King, E.V., Conley, D., 2018. Wave and tidal controls on embayment circulation and headland bypassing for an exposed, macrotidal site. J. Mar. Sci. Eng. 6, 94
Moltmann, T., Turton, J., Zhang, H., Nolan, G., Gouldman, C., Griesbauer, L., et al., 2019. A global ocean observing system (GOOS), delivered through enhanced collaboration across regions, communities, and new technologies. Front. Mar. Sci.doi: 10.3389/ fmars.2019.00291.
Oliver, T.S.N., Tamura, T., Hudson, J.P., Woodroffe, C.D., 2017. Integrating millennial and interdecadal shoreline changes: Morpho-sedimentary investigation of two prograded barriers in southeastern Australia. Geomorphology 288, 129–147.
Pacheco, A., Horta, J., Louriero, C., Ferriera, O., 2015. Retrieval of nearshore bathymetry from Landsat 8 images: a tool for coastal monitoring in shallow waters. Remote Sens. Env. 159, 102–116.
Reeve, D.E., Karunarathna, H., Pan, S., Horrillo-Caraballo, J.M., Róży ski, G., Ranasinghe, R., 2016. Data-driven and hybrid coastal morphological prediction methods for mesoscale forecasting. Geomorphology 256, 49–67.
Reeve, D.E., Horrillo-Caraballo, J., Karunarathna, H., Pan, S., 2019. A new perspective on meso-scale shoreline dynamics through data-driven analysis. Geomorphology 341, 169–191.
Salcedo-Sanz, S., Nieto Borge, J.C., Carro-Calvo, L., Cuadra, L., Hessner, K., Alexandre, E., 2015. Significant wave height estimation using SVR algorithms and shadowing information from simulated and real measured X-band radar images of the sea surface. Ocean Eng. 101, 244–253.
Short, A.D., Fotheringham, D.G., 1986. Coastal morphodynamics and Holocene evolution of the Kangaroo Island coast, South Australia. Technical Report No. 86/1, Coastal Studies Unit, University of Sydney, Sydney, 112 pp.
Short, A.D., Hesp, P.A., 1982. Wave, beach and dune interactions in southeast Australia. Mar. Geol. 48, 259–284.
Short, A.D., 2006. Australian beach systems – nature and distribution. J. Coastal Res. 22, 11–27
Short, A.D., Jackson, D.W.T., 2013. Beach morphodynamics. In: John F. Shroder, Eds., Treatise on Geomorphology. Elsevier, Academic Press, Amsterdam, San Diego, pp. 106–129. ISBN 9780123747396.
Simmons, J.A., Splinter, K., Beuzen, T., 2019. Data-driven modelling of shoreline evolution. Coastal Sediments 2019, pp. 733–741.
Sonu, C.J., 1972. Field observations of nearshore circulation and meandering currents. J. Geophys. Res. 77, 3232–3247
Sonu, C.J., 1973. Three dimensional beach changes. J. Geol. 81, 42–64
Sonu, C.J., Murray, S.P., Hsu, S.A., Suhayda, J.N., Waddell, E., 1973. Sea breeze and coastal processes. Trans. Amer. Geophys. Union 54, 820–833
Splinter, K.D., Turner, I.L., Davidson, M.A., Barnard, P., Castelle, B., Oltman-Shay, J., 2014. A generalized equilibrium model for predicting daily to interannual shoreline response. J. Geophys. Res: Eart. Surf. 119 (9), 1936–1958
Splinter, K.D., Harley, M.D., Turner, I.L., 2018. Remote sensing is changing our view of the coast: insights from 40 years of monitoring at narrabeen-collaroy. Australia. Remote Sens. 10, 1744
Stringari, H.E., Power, H., 2019. The Fraction of Broken Waves in Natural Surf Zones. J. Geophys. Res.: Oceans, https://doi.org/10.1029/2019JC015213
Turner, I.L., Goodwin, I.D., Davidson, M.A., Short, A.D., Pritchard, T.R., Lane, C., Cameron, D.W., MacDonald, T., Middleton, J., Splinter, K.D., 2011. Planning for an Australian National Coastal Observatory: monitoring and forecasting coastal erosion in a changing climate. Australasian Coast and Ports Conference, Perth, Institute of Engineers Australia, 7 pp.
Wright, L.D., Coleman, J.M., 1971. Variation in morphology of major river deltas as functions of ocean wave and river discharge regimes. Amer. Assoc. Petrol. Geol. 57, 370–398
Wright, L.D., Chappell, J., Thom, B.G., Bradshaw, M.P., Cowell, P.J., 1979. Morphodynamics of reflective and dissipative beach and inshore systems. Southeastern Australia. Mar. Geol. 32, 105–140.
Wright, L.D., Thom, B.G., 1977. Coastal depositional landforms: a morphodynamic approach. Prog. Phy. Geogr. 1, 412–459.
Yunus, A.O., Dou, J., Song, X., Avtar, R., 2019. Improved bathymetric mapping of coastal and lake environments using sentinel-2 and landsat-8 images. Sensors 19 (12), 2788.
Beach sand and its origins
Burghard Flemming Senckenberg Institute, Suedstrand, Wilhelmshaven, Germany
Chapter outline
2.1 Introduction 15
2.2 Brief history of provenance studies 17
2.3 Sources of beach sand 19
2.3.1 Fluvial sand supply 19
2.3.2 Production of sand by coastal erosion 21
2.3.3 Production of sand by seabed erosion 21
2.3.4 Biological production of carbonate sands 22
2.3.5 Artificial and nourished beaches 23
2.4 Methodological aspects 24
2.4.1 Sampling strategies and grain-size analysis 24
2.4.2 Hydraulics of beach sand 27
2.4.3 Grain size and beach slope relations 27
2.5 Conclusions and recommendations 32 References 32
2.1 Introduction
Based on a detailed analysis of satellite images, a quantitative estimate of the global occurrence of sandy beaches suggests that about 31% of the world’s shorelines consist of sandy beaches (Luijendijk et al., 2018). Of these, 24% are eroding, 28% are accreting and 48% are stable. Viewed in a global context, the African shoreline boasts the highest proportion of sandy beaches (66%), whereas Europe’s coastline has the smallest proportion (22%). As the origins of beach sand are manifold, it is useful to distinguish between magmatic and sedimentary source rocks and their mineral compositions. Igneous rocks, that is, intrusive and extrusive magmatic rocks, are the original primary sources of sand worldwide. Fig. 2.1 shows that the mineral content of most intrusive rocks is mirrored in an extrusive counterpart. For example, granite (an intrusive rock) and rhyolite (an extrusive rock) have, by definition, identical volumetric mineral compositions (40% potash feldspar, 15% plagioclase feldspar, 27% quartz, 12% mica and 6% amphiboles). The only difference between the two is the size of the minerals (Clark, 1966). Thus, intrusive rocks are composed of relatively large minerals because of slow cooling, whereas those of the rapidly cooling extrusive rocks are much smaller. Metamorphic rocks, which comprise sedimentary rocks subjected to high temperatures and partial melting, are composed of minerals spanning larger grain-size spectra.
Sandy Beach Morphodynamics. http://dx.doi.org/10.1016/B978-0-08-102927-5.00002-3
Copyright
Figure 2.1 Mineral composition of intrusive magmatic rocks and their extrusive counterparts. Source: Modified after Clark, 1966; Flemming, 2011.
Sedimentary rocks, a major secondary source of sand, can roughly be divided into marine volcaniclastics (∼15.0% by volume), continental pyroclastics (∼2.6%), graywackes (∼2.6%), arkoses (∼5.3%), quartz sandstones (∼7.5%), mudrocks (∼60%), dolomites (∼0.7%), limestones (∼5.3%) and evaporites (∼1.0%) (Ronov, 1964; Garrels and Mackenzie, 1971). Although most sedimentary rocks incorporate variable amounts of fine-grained matrix material, pyroclastics, sandstones (including graywackes, arkoses, quartzites) and limestones are the most prominent sedimentary
sources of sand. Carbonate rocks have either been derived directly by precipitation of CaCO3 from waters supersaturated in carbonate or by the accumulation of bioclastic material produced by marine organisms (Bathurst, 1971; Milliman, 1974). In most cases beach sands derived from unconsolidated or semi-consolidated terrestrial deposits or from sedimentary rocks have undergone several cycles of erosion, transportation and deposition (Veevers, 2015).
From the above, it is clear that from the nature and composition of igneous and sedimentary rocks exposed within river catchments, or along rocky shorelines and shore platforms, it is possible to roughly estimate overall grain sizes and mineral compositions of sediments delivered to the beaches, although estimates of overall volume or mass fluxes remain problematical. Only in special circumstances have sediment yields and/or mineral compositions of coastal deposits been successfully estimated (Gómez-Pujol et al., 2013; Gregoire et al., 2017; Flemming and Martin, 2018). In this context, it must be borne in mind that different minerals have different resistances to mechanical abrasion (Thiel, 1940; Suttner, 1989), or different responses to hydraulic transport (Rittenhouse, 1943; Bagnold, 1968). Thus, quartz grains have the greatest resistance to mechanical wear and tear, whereas feldspars, because of their perfect cleavage, are easily broken down into increasingly smaller fragments when exposed to mechanical forces. As a consequence, quartz grains tend to be enriched relative to less-resistant minerals, especially in the course of prolonged fluvial transport. However, close to their source, even less-resistant minerals may form dominant sedimentary components. For example, olivine, a relatively unstable mineral, often occurs in abundance on beaches of volcanic islands, where it can be recognised by the characteristic olive-coloured hue of the sand.
In spite of the considerable variability in the amount and type of sediment supplied to the coastal zones of the world, there appears to be a distinct latitudinal trend in the average composition of shelf and nearshore sediments (Fig. 2.2). It follows that this trend should, at least in part, be reflected in the composition of beach sediments of adjacent coasts (Hayes, 1967).
2.2 Brief history of provenance studies
An early example of systematic recordings of beach sediment types is the instruction of Rear Admiral Sir Francis Beaufort (1774–1857), hydrographer of the British Admiralty from 1829 to 1846, to the captains under his command in 1831: “The nature of the shore, whether high cliff, low rock or flat beach, is of course inserted on every survey – but much more may be easily and usefully expressed – for instance the general elevation of the cliffs and their colour, the material of the beach, mud, sand, gravel or stones, etc.” (cited in Wilford, 2001). Shortly after, in 1833, Friedrich Arends, a German geographer and perhaps the first person to concern himself scientifically with, amongst others, the nature of coastal deposits, published a remarkable account of historical storm surge records dating back to 800 AD and the nature of the seabed and the shores lining the German sector of the North Sea (Arends, 1833).
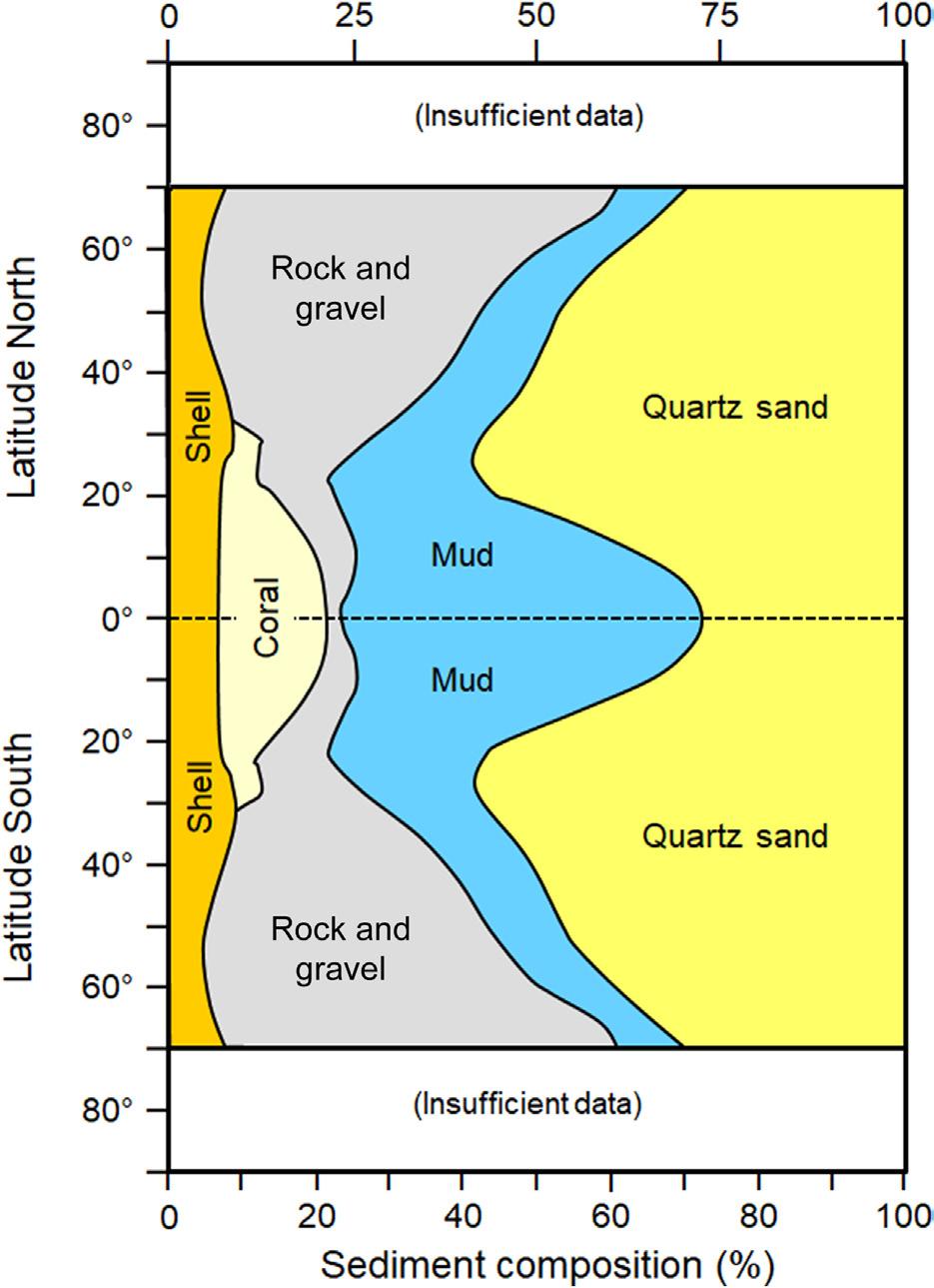
2.2 Latitudinal distribution of sediments and rocks on continental shelves with implications for beach sediment composition.
Source: Modified after Hayes, 1967; Flemming, 2011.
North American studies specifically dealing with sea beaches and the relative importance of various sediment sources were carried out as early as 1898 (Cornish, 1898) and 1904 (Barrell, 1904).
The modern era of beach research can be said to have begun in 1919 with the comprehensive treatment of shore processes by Douglas Wilson Johnson (Johnson, 1919).
In the early 1920s Wentworth (1922) introduced a first systematic scale grading of sediments on the basis of the negative binary logarithm (–log2) of grain sizes measured in millimetres, a classification still widely in use today. Up to the 1950s, dedicated source studies of beach sands were conducted at various localities along the east coast of the USA (MacCarthy, 1931; Colony, 1932; Martens, 1935, 1939), along the Gulf Coast of Texas (Bullard, 1942) and the coast of California (Reed, 1930; Trask, 1952). From the 1950s onwards, the study of continental, coastal and marine depositional sedimentary environments literally exploded and, within that context, the question of sediment provenance played a crucial role (Basu, 2003). This is
Figure
reflected by the proliferation of books (Ibbeken and Schleyer, 1991) and especially conference proceedings (Zuffa, 1985; Morton et al., 1991; Johnsson and Basu, 1993; Valloni and Basu, 2003) dealing with this issue at global and regional scales. A relatively recent review with numerous relevant citations can be found in Weltje and von Eynatten (2004). Furthermore, besides numerous coastal engineering conferences held bi-annually since 1950 under the acronym ICCE (e.g. Lynett, 2016), there have since 1977 also been nine conferences – since 1999 at four-yearly intervals – that were specifically dedicated to ‘Coastal Sediments’ in the widest sense (e.g. Wang et al., 2015).
2.3 Sources of beach sand
2.3.1
Fluvial sand supply
Since the 1980s, the annual global sediment yield via terrestrial drainage was variably estimated to range between 13.5 × 109 t yr 1 (Milliman and Meade, 1983) and 15–20 × 109 t yr 1 (Walling and Webb, 1983, 1996). In the 1990s, several authors argued that, due to human-induced soil losses, these estimates needed upgrading to 50 × 109 t yr 1 (Oldeman et al., 1991) or even 75 × 109 t yr 1 (Pimentel et al., 1995). The most recent discourse on the global sediment flux to the world’s oceans, differentiated by climatic setting, can be found in Syvitski et al. (2003). The large variability of annual sediment yields estimated by various research groups is an indication that further in-depth research is required in this respect, especially also focusing on the partitioning of sediment yields into mineral and grain-size compositions. Various approaches in this context have recently been suggested by Brown et al. (2009), and a good summary of the current state of knowledge in the prediction of regional sediment yields has been summarised by De Vente et al. (2013).
Besides the classical white or yellowish coloured quartz sands (specific density δ = 2.65) of which most beaches worldwide are composed, almost black-coloured beaches can be found along the shores of volcanic islands not fringed by coral reefs. Two examples from Tenerife (Canary Islands, Spain) are illustrated in Fig. 2.3. Some island pocket beaches may be almost entirely composed of black volcaniclastic sand (Fig. 2.3A), whereas in other places the beaches may be composed of variable mixtures of volcaniclastic and bioclastic material, giving the sand a freckled appearance (Fig. 2.3B). While the volcaniclastic sand is mainly a product of weathered and eroded onshore volcanic rocks, tuffs and ashes, the bioclastic components are of marine (intertidal and shallow subtidal) origin, being transported to the beach by wave-driven onshore or alongshore currents before becoming mixed with the volcaniclastic sands.
Other exotic minerals mainly supplied to the coast by rivers are so-called heavy minerals, which have specific densities of δ > 2.9 (Table 2.1). In most cases the proportion of heavy minerals is very small relative to the amount of quartz grains, and hydraulic sorting thus commonly produces dark heavy mineral laminations along bedding planes in deposits otherwise dominated by quartz grains. However, where the