Recent Trends in Carbohydrate Chemistry
Synthesis and Biomedical Applications of Glycans and Glycoconjugates
Volume 2
Edited by
Amélia Pilar Rauter
Bjørn
E. Christensen
László
Somsák
Paul
Kosma
Roberto
Adamo
Elsevier
Radarweg 29, PO Box 211, 1000 AE Amsterdam, Netherlands
The Boulevard, Langford Lane, Kidlington, Oxford OX5 1GB, United Kingdom 50 Hampshire Street, 5th Floor, Cambridge, MA 02139, United States
© 2020 Elsevier Inc. All rights reserved.
No part of this publication may be reproduced or transmitted in any form or by any means, electronic or mechanical, including photocopying, recording, or any information storage and retrieval system, without permission in writing from the publisher. Details on how to seek permission, further information about the Publisher’s permissions policies and our arrangements with organizations such as the Copyright Clearance Center and the Copyright Licensing Agency, can be found at our website: www.elsevier.com/ permissions.
This book and the individual contributions contained in it are protected under copyright by the Publisher (other than as may be noted herein).
Notices
Knowledge and best practice in this field are constantly changing. As new research and experience broaden our understanding, changes in research methods, professional practices, or medical treatment may become necessary.
Practitioners and researchers must always rely on their own experience and knowledge in evaluating and using any information, methods, compounds, or experiments described herein. In using such information or methods they should be mindful of their own safety and the safety of others, including parties for whom they have a professional responsibility.
To the fullest extent of the law, neither the Publisher nor the authors, contributors, or editors, assume any liability for any injury and/or damage to persons or property as a matter of products liability, negligence or otherwise, or from any use or operation of any methods, products, instructions, or ideas contained in the material herein.
Library of Congress Cataloging-in-Publication Data
A catalog record for this book is available from the Library of Congress
British Library Cataloguing-in-Publication Data
A catalogue record for this book is available from the British Library
ISBN: 978-0-12-820954-7
For information on all Elsevier publications visit our website at https://www.elsevier.com/books-and-journals
Publisher: Susan Dennis
Acquisitions Editor: Emily M. McCloskey
Editorial Project Manager: Kelsey Connors
Production Project Manager: Omer Mukthar
Cover Designer: Victoria Pearson
Typeset by SPi Global, India
Contributors
Sara Ali Leiden Institute of Chemistry, Leiden University, Leiden, Netherlands
Francesca Berni Leiden Institute of Chemistry, Leiden University, Leiden, Netherlands
Francesco Berti GSK Vaccines, Technical R&D, Siena, Italy
Ralph Biemans GSK Vaccines, Rixensart, Belgium
Omar Boutureira Department of Analytical Chemistry and Organic Chemistry, Rovira i Virgili University, Tarragona, Spain
Maude Cloutier Institut national de la recherche scientifique (INRS), Laval, QC, Canada
Jeroen D.C. Codée Leiden Institute of Chemistry, Leiden University, Leiden, Netherlands
Paolo Costantino GSK, Siena, Italy
Jacopo Enotarpi Leiden Institute of Chemistry, Leiden University, Leiden, Netherlands
Charles Gauthier Institut national de la recherche scientifique (INRS), Laval, QC, Canada
Paul Kosma Department of Chemistry, University of Natural Resources and Life Sciences Vienna, Vienna, Austria
Paul Messner Department of NanoBiotechnology, NanoGlycobiology Unit, Universität für Bodenkultur Wien, Vienna, Austria
Francesca Micoli GSK Vaccines Institute for Global Health (GVGH), Siena, Italy
Kevin Muru Institut national de la recherche scientifique (INRS), Laval, QC, Canada
Laura Polito CNR-SCITEC, Milan, Italy
x Contributors
Neil Ravenscroft Department of Chemistry, University of Cape Town, Rondebosch, South Africa
Maria Rosaria Romano GSK Vaccines srl, Siena, Italy
Christina Schäffer Department of NanoBiotechnology, NanoGlycobiology Unit, Universität für Bodenkultur Wien, Vienna, Austria
Sebastian Strobl Department of Chemistry, University of Natural Resources and Life Sciences, Vienna, Austria
Miguel A. Valvano Wellcome-Wolfson Institute for Experimental Medicine, Queen’s University Belfast, Belfast, United Kingdom; Department of Microbiology and Immunology, University of Western Ontario, London, ON, Canada
Gijs A. van der Marel Leiden Institute of Chemistry, Leiden University, Leiden, Netherlands
Alla Zamyatina Department of Chemistry, University of Natural Resources and Life Sciences, Vienna, Austria
Preface
In this volume, the important contribution of carbohydrate chemistry for the generation of complex biomolecules, namely glycolipids, glycoproteins, and glycans, is illustrated. These biomolecules play pivotal roles in many biological processes, particularly in the establishment of infections, as they contribute to host-pathogen interactions and cell invasion, as well as to the evasion mechanisms utilized by pathogens to overcome the immunological defense of the host.
Part 1 starts with an outline of prokaryotic glycoproteins focusing on their functional roles known to date. The following chapter illustrates ligation reactions to give lipid-linked glycans anchored to bacterial cytoplasmic membrane, in which the glycan residues in extracytoplasmic loops are important for substrate recognition and catalysis. These two chapters enlighten the significant role of glycans in infection, which has motivated carbohydrate chemists to investigate synthetic approaches to glycan and glycoconjugate molecular entities. The next chapters cover elegant syntheses of glycolipids and lipopolysaccharides, of oligosaccharides that are virulent factors in infection by pathogenic bacteria, and of teichoic acids.
Glycoconjugate vaccines became a successful strategy to prevent infection but many infectious diseases still need to be addressed. The enormous efforts in the area of glycoconjugate vaccines, can be witnessed in part 1 and part 2 chapters on vaccines, covering synthesis and analysis of carbohydrate-based vaccines, modern methodologies for their preparation and generation of glyconanoparticles.
We hope that Recent Trends in Carbohydrate Chemistry will pay the due tribute to carbohydrate chemistry that generates innovation of the scientific knowledge, and offers the tools to access complex molecules that are, indeed, the key for developing diagnostics and new efficient therapeutics for many of the incurable diseases threatening the human being.
Amélia Pilar Rauter
Bjørn E. Christensen László Somsák Paul Kosma Roberto Adamo
Prokaryotes: Sweet proteins do matter
Christina Schäffer, Paul Messner Department of NanoBiotechnology, NanoGlycobiology Unit, Universität für Bodenkultur Wien, Vienna, Austria
Chapter outline
1 Introduction 3
2 Cell surface (S-) layer glycoproteins 5
3 Bacteria 6
3.1 Lactic acid bacteria 6
3.2 Oral pathogens 9
3.3 Other bacteria 12
3.4 Archaea 14
4 Flagella/Archaella and fimbriae 17
4.1 Bacteria 17
4.2 Archaea 19
5 Adhesins, bacteriocins, invasins, and glycocins 19
5.1 Streptococcus gordonii 19
5.2 Streptococcus salivarius 20
5.3 Streptococcus agalactiae 20
5.4 Streptococcus mutans 21
5.5 Staphylococcus aureus 21
5.6 Enterococcus durans 22
5.7 Lactobacillus plantarum 22
5.8 Lactobacillus casei 23
5.9 Xanthomonas citri 23
5.10 Escherichia coli 23
5.11 Actinoplanes sp. 24
5.12 Trichomonas vaginalis 24
6 Spores 25
6.1 Bacillus anthracis 25
7 Concluding remarks 25 References 27
1 Introduction
Glycoproteins are among the most variable molecules of life, which explains their manifold functions and simultaneously makes them pivotal subjects of research. When displayed at the prokaryotic cell surface, or as cell appendages, glycoconjugates translate
into a cell- or strain-specific barcode, mediating dedicated interactions with the cellular environment and the host, and, overall, increasing prokaryotic fitness in their niche. Thus, the potential of these molecules for designing novel anti-infective therapies has been appreciated during the past decade, and much research in this direction is on the way.
For prokaryotic organisms (both archaea and bacteria), protein glycosylation is a major posttranslational modification (PTM) that holds the important capability to modulate protein function, thereby influencing the organisms’ physiology.1–4 Despite the simplicity of their cellular architecture in comparison to eukaryotes, prokaryotes display a much wider variety of protein glycosylation with regard to carbohydrate components, noncarbohydrate substituents, glycosidic linkages, structures, and protein–carbohydrate linkages including N-, O-, and S-glycosylation (Fig. 1), with many features still to be uncovered. Strikingly, most glycoproteins are synthesized by similar biosynthesis mechanisms. Prokaryotes produce their major glycoconjugates by using either a sequential or an en bloc mechanism—in analogy to what is well known from lipopolysaccharide (LPS) biosynthesis8—with both options coexisting in many species for different macromolecules.9
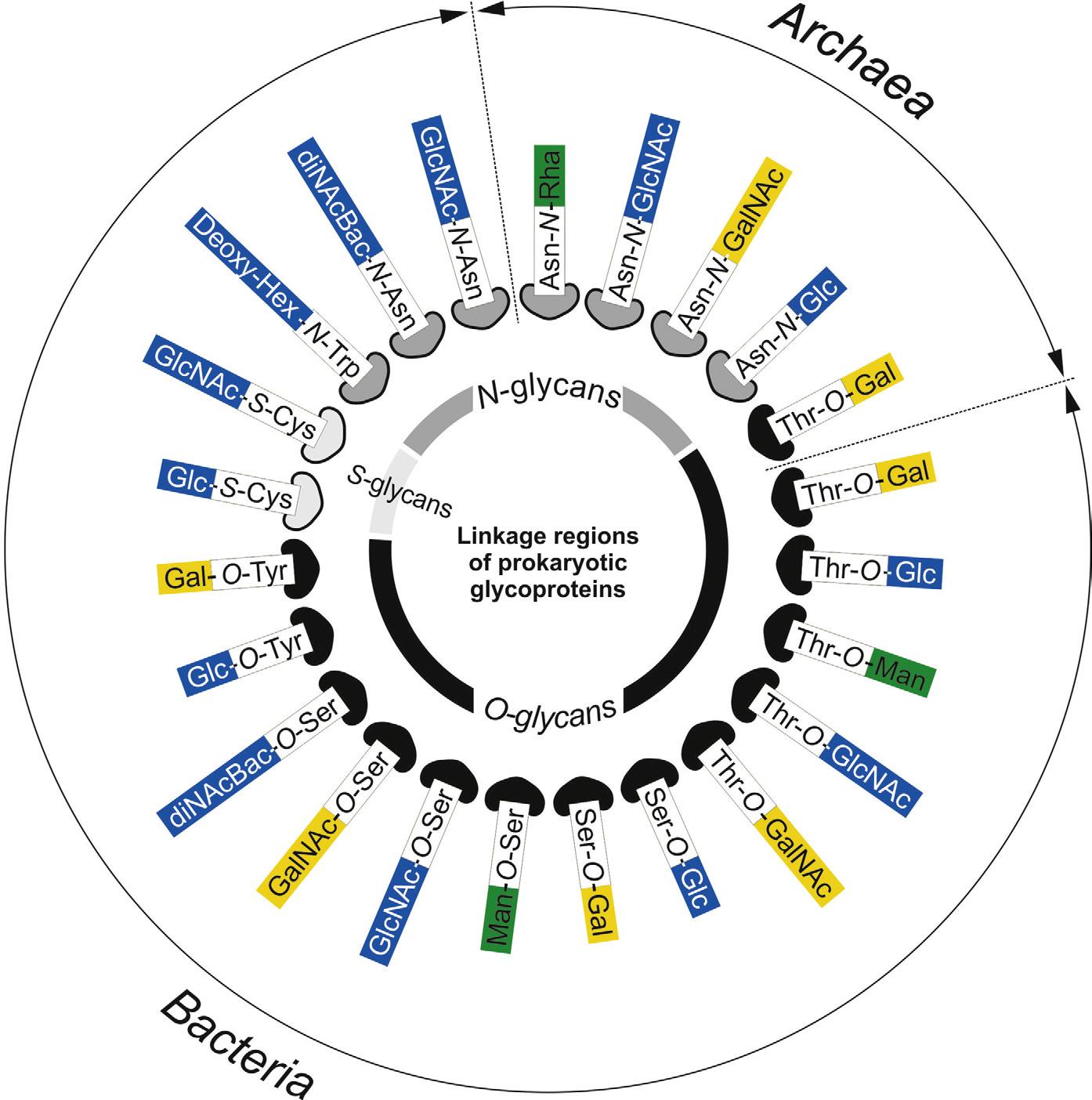
Knowledge of the structures and understanding of the biosynthesis of the different bacterial glycoconjugates is important for developing effective “metabolic glycoengineering” (MGE) strategies in the future.10,11 The occurrence, biosynthesis, and possible functions of prokaryotic glycoproteins are increasingly documented for pathogens,5,12 but, yet, they are not widely described for beneficial bacteria, such as probiotic bacteria.13,14 Overall, from a scientific point of view, sufficient foundation is available to support the applications of MGE in bacteria based on methods to preferentially direct metabolic intermediates into pathways involved in LPS, peptidoglycan (PGN), teichoic acid, bacterial appendages, and capsule polysaccharide production.15 MGE strategies ranging from initiating an immune response against carbohydrate antigens associated with pathogenic microbes to disrupting their ability to adhere to mammalian cell surfaces, or using bacteria as hosts for recombinant glycoprotein production are envisaged.
In this chapter, prokaryotic glycoproteins are described according to their cellular localization. We are particularly focusing on research from the past three years; for a comprehensive overview on prokaryotic glycoproteins until then, the reader is referred to our recent review.5
2 Cell surface (S-) layer glycoproteins
It has been more than 65 years since Houwink showed by electron microscopy a monolayer of regularly arrayed macromolecules on the external surface of the cell wall of an unidentified Spirillum strain16—a cell surface structure that is nowadays commonly known as cell surface layer (S-layer). S-layers are ubiquitous in bacteria and archaea—with an ever-increasing number of species being added to the list of S-layer carrying organisms—where they account for the most abundant cellular proteins.17,18 The most frequent modification of S-layers is glycosylation—for bacteria, so far in all but one case O-linked protein glycosylation has been reported,5,19,20 while in archaea, both O- and N-glycans seem to occur with comparable frequency.21,22 S-layers are formed by the intriguing self-assembly capability of constituting S-layer (glyco)proteins into “two-dimensional crystalline lattices” with hexagonal (p6), tetragonal (p4), trimeric (p3), and oblique (p2) symmetry.23 In the native habitat, S-layers contribute to the structural integrity of the organisms’ cell envelope, provide environmental protection, such as protection against bacteriophages and phagocytosis, low pH and high-molecular-weight substances (e.g., lytic enzymes),24 and can be involved in the mediation of adhesion and virulence. In contrast, S-layers might be lost under laboratory cultivation conditions, probably due to the absence of a selective pressure.17 S-layers form a closed monolayer on the bacterial cell surface during all stages of the bacterial growth cycle. Thus, the maintenance of the S-layer depends on the stable and flexible attachment to continuously changing cell surfaces. For this purpose, dedicated noncovalent attachment strategies have developed during bacterial evolution. These involve S-layer homology (SLH) domains or protein regions rich in positively charged amino acids. These can be located either at the N- or at the C-terminus of the S-layer protein and recognize a PGN-associated secondary cell wall polymer (SCWP) as a cell wall ligand.25–30 A substantial body of knowledge has accumulated over the
past decades on cell wall anchoring, assembly properties, chemistry, biosynthesis, and three-dimensional structure of S-layer (glyco)proteins, as well as on their biotechnological application potential, which is mainly based on the nanometer-scale periodicity of these unique self-assembly structures.31–36
3 Bacteria
3.1 Lactic acid bacteria
Lactic acid bacteria constitute a diverse group of “generally regarded as safe” (GRAS) microbes that are not only primarily used as starter cultures and probiotics, but also being developed as production systems in industrial biotechnology for biocatalysis and transformation of renewable feedstocks to commodity and high-value chemicals and health products.36–38
The widely used commercial probiotic species Lactobacillus acidophilus (Lb. acidophilus) produces an S-layer protein (SlpA).39 S-layers frequently coat the cell surface of lactobacilli that are taxonomically assigned to the Lb. acidophilus group A, including Lb. acidophilus, Lactobacillus helveticus, Lactobacillus crispatus, Lactobacillus amylophilus, Lactobacillus gallinarum, Lactobacillus jensenii, Lactobacillus kefiranofaciens, and Lactobacillus amylovorus Lactobacillus S-layer proteins elicit physiological and immunological responses and show potential for use as antigen carriers in live oral vaccine design because of their adhesive and immunomodulatory properties, as well as their GRAS status.40 Certain probiotic Lactobacillus species produce S-layers with embedded, loosely associated proteins (SLAPs). In Lb. acidophilus 20–40 SLAPs have been found.14,41 It is assumed that both S-layer proteins and SLAPs represent a collection of proteins that likely elicit common processes, such as interacting with mucosal tissues and exerting significant physiological, enzymatic, and immunological consequences.14,42
Some Lactobacillus S-layer proteins are glycosylated. Basic knowledge of protein glycosylation in these bacteria is pivotal not only for our understanding about their repertoire of glycan-mediated interactions but also for MGE approaches in food-grade microbes such as Lactobacillus rhamnosus GG, whose major secreted protein Msp1 is glycosylated with Concanavalin A (ConA)-reactive sugars at serine residues.13 Lb. acidophilus ATCC 4365 S-layer protein failed to bind C-type lectin and, thus, is probably not glycosylated.43
3.1.1 Lactobacillus buchneri
The recently sequenced Lb. buchneri strains CD03444,45 and 41021/25146 were shown to possess a glycosylated S-layer protein SlpB that self-assembles into an oblique lattice. Biochemical and mass spectrometric (MS) analyses revealed that SlpB is the most abundant protein in Lb. buchneri CD034 and that it is O-glycosylated at four serine residues within the sequence Ser152-Ala-Ser154-Ser155-Ala-Ser157 with each possessing, on average, seven α1,6-linked glucose residues (Fig. 2). Protein
Fig. 2 Schematic depiction of selected S-layer glycan structures from bacteria (left) and archaea (right) to illustrate the diversity of prokaryotic protein-bound glycans. Abbreviations: Ac, CH3CO; CONH2, amidyl-; Me, CH3 . Monosaccharide symbols according to the Symbol Nomenclature for Glycans (SNFG)47. Lb. buchneri, 45,46 Ta. forsythia, 48,49 Pa. alvei, 50,51 Hfx. volcanii, 52–56 and Su. acidocaldarius. 57
glycosylation analysis was extended to strain Lb. buchneri NRRL B-30929, where an analogous glucosylation scenario was found, with the S-layer glycoprotein SlpN containing an O-glycosylation motif identical to that of SlpB.58 This corroborated previous data on S-layer protein glucosylation of strain 41021/251 and led to the proposal of a species-wide S-layer protein O-glucosylation in Lb. buchneri targeted at the sequence motif SASSAS.45
3.1.2 Lactobacillus kefiri
The S-layer protein of another member of the Lb. buchneri group, 59 Lb. kefiri, revealed interesting strain-specific features.20 Proteomic and genomic approaches were used to gain knowledge about the sequences of the S-layer protein encoding genes of six aggregative and 16 nonaggregative, potentially probiotic, Lb. kefiri strains. Peptide mass fingerprint analysis confirmed the identity of S-layer proteins extracted from Lb. kefiri, and primers based on the homology with phylogenetically related species were used to amplify the S-layer genes. The total length of the mature proteins from
10 selected strains varied from 492 to 576 amino acids, all S-layer proteins have calculated pI values between 9.4 and 9.6, and contain the canonical O-glycosylation site SASSAS. The N-terminal region is relatively conserved and shows a high percentage of positively charged amino acids. Major differences among these S-layer proteins are found in the C-terminal region. Different Lb. kefiri groups could be distinguished based on the similarities observed in the peptide mass fingerprint spectra of the mature S-layer proteins. Interestingly, S-layer proteins of the collective strains are 100% homologous, although these strains were isolated from different kefir grains. This knowledge provides relevant data for a better understanding of the mechanisms involved in S-layer protein functionality and could contribute to the development of products of biotechnological interest from potentially probiotic bacteria.20
The S-layer protein of Lb. kefiri CIDCA 83111 60 has a more complex glycosylation pattern when compared to Lb. buchneri S-layer proteins. 45 MALDI-TOF MS (matrix-assisted laser desorption/ionization-time-of-flight mass spectrometry) analysis confirmed the presence of oligosaccharides composed of glucose (Glc) units carrying galacturonic acid (GalA). Further, nano-HPLC-ESI (highperformance liquid chromatography-electrospray ionization) analysis of the glycopeptides showed two O -glycosylated peptides. This includes the peptide sequence SSASSASSA already identified as a signature glycosylation motif in Lb. buchneri , which is substituted on average with eight Glc residues and decorated with GalA, and another O -glycosylated site on peptide 471–476, with a Glc 5-8GalA 2 structure. As 10 characteristic sequons (Asn-X-Ser/Thr) are present in the S-layer amino acid sequence, PNGase F digestion was performed to release putative N-linked oligosaccharides. Anion exchange chromatography analysis showed mainly short N -linked chains. NanoHPLC-ESI in the positive and negative ion modes were useful to determine two different peptides substituted with short N -glycan structures. This is the first description of N -glycans in S-layer glycoproteins from Lactobacillus species. 60
A detailed characterization of Lb. kefiri S-layer protein glycosylation is essential to establish the basis for investigating and understanding its biological role. It is known that S-layer proteins from kefir-isolated Lb. kefiri strains are involved in the interaction of bacterial cells with yeasts present in kefir grains and are also capable of antagonizing the adverse effects of different enteric pathogens. Therefore, characterization of type and site of glycosylation of the S-layer protein may help to understand these important properties.60 The role of the Lb. kefiri S-layer glycans was investigated for their internalization by murine macrophages as well as immunomodulatory capacity and effect on LPS-stimulated macrophages.20 RAW 264.7 cells internalized the Lb. kefiri CIDCA 8348 S-layer protein in a process that was mediated by carbohydrate-receptor interactions, as concluded from the inhibition with glucose (Glc), mannose (Man), or EGTA [3,12-bis(carboxymethyl)-6,9-dioxa3,12-diazatetradecane-1,14-dioic acid, named as Ethylene Glycol bis(aminoethylether)-N,N,N′,N′ , Tetra-Acetic acid] as Ca2 + chelating agent. These results correlated with the recognition of the S-layer protein by ConA. Further, it was shown that while the S-layer protein did not activate macrophages by itself, it favored the LPS-induced response, as concluded from a significant increase in the expression of the surface
cell markers MHC-II, CD86, and CD40, as well as in IL-6 and IL-10 expression at both transcript and protein levels, in comparison with cells stimulated with LPS alone. The presence of EGTA completely abrogated the synergistic effect between LPS and S-layer glycoproteins. These results strongly suggest the involvement of both glycan residues and Ca2 + ions in the recognition of the Lb. kefiri S-layer protein by cellular receptors on murine macrophages. Moreover, these results suggest a potential of this glycoprotein for the development of new adjuvants capable of stimulating antigen presenting cells by interaction with glycan receptors.20
Lactobacilli are extensively exploited as cell surface display systems for applied purposes,61,62 however, little is known about how the S-layer as a natural cell surface display matrix attached to the Lactobacillus cell envelope. It was investigated whether a cell wall glycopolymer serves as a cell wall ligand in a scenario comparable to that found for S-layer carrying Bacillaceae , where SCWPs serve as cell wall ligands. 62 Lipoteichoic acid was isolated from the Lb. buchneri CD034 44 cell wall as a significant fraction of the bacterium’s cell wall glycopolymers, structurally characterized, and analyzed for its potential to mediate binding of the endogenous S-layer protein SlpB to the cell wall. 63 Combined compositional analyses and nuclear magnetic resonance (NMR) spectroscopy revealed the lipoteichoic acid 64 to be composed of on average 31 glycerol-phosphate repeating units partially substituted with α - d -glucose, and with a Gro-(PGro*-P) ∼31-6- α - d -Gal p (1 → 2)- αd -Glc p (1 → 3)-1,2-diacyl- sn -Gro (*H or ∼ 30% α - d -Glc p ) glycolipid anchor. The specificity of binding between recombinant Lb. buchneri CD034 S-layer protein rSplB and purified lipoteichoic acid was obtained by single-molecule force spectroscopy, revealing an interaction force of about 45 pN between these components. Thus, lipoteichoic acid could be identified as a cell wall ligand for the Lb. buchneri CD034 S-layer protein.63 However, the binding region on the S-layer protein remains to be determined.
For the S-layer proteins of Lb. acidophilus ATCC 435665 and Lb. crispatus,66 a C-terminal cell wall targeting region was identified. The highly basic nature of the S-layer (pI >9.5) seems to be involved in electrostatic interactions with DC-SIGN (dendritic cell-specific intercellular adhesion molecule-3-grabbing nonintegrin), since high pH abolished the inhibitory effect on infection caused by the S-layer. In silico analysis predicts a Ca2 +-dependent carbohydrate recognition domain in the SlpA protein. This feature of this probiotic S-layer contributes to pathogen exclusion and may be applied as an antiviral agent to inhibit several kinds of viruses.43
3.2 Oral pathogens
Research on glycoproteins from oral pathogens has developed only during the past decade and our current picture is mainly derived from studies on Tannerella forsythia (Ta. forsythia) and, to a lesser extent, Porphyromonas gingivalis (Po. gingivalis). Together with Treponema denticola these organisms constitute the so-called red complex of bacteria that is crucially involved in the etiology of periodontitis. Periodontitis is a biofilm-caused infectious disease and the most frequent inflammatory disease of bacterial origin worldwide.67
3.2.1 Tannerella forsythia
Ta. forsythia is an anaerobic, Gram-negative periodontal pathogen whose S-layer, among several other factors,68 was shown to be a virulence factor,69,70 and to modulate the host immune response.71–74 A unique O-linked, complex oligosaccharide decorates the S-layer as well as other cell surface proteins of the bacterium48,49 (Fig. 2). Another identified glycoprotein is the cell wall and secreted Bacteroides surface protein A (BspA)75 which is also a virulence factor.68 The S-layer has a square lattice symmetry and is composed of the two S-layer glycoproteins TfsA and TfsB,76 which are secreted via a type IX secretion system that is decoupled from protein O-glycosylation.77,78
Recently, biosynthesis of nonulosonic acids (NulO) present at the terminal position of this glycan was investigated.79 The genome of Ta. forsythia type strain ATCC 43037 encodes a gene locus for the synthesis of pseudaminic acid (Pse), while strains FDC 92A2 and UB4 possess a locus for the synthesis of legionaminic acid (Leg) instead. In contrast to the NulO in ATCC 43037, which was identified as a Pse derivative (5-N-acetimidoyl-7-N-glyceroyl-3,5,7,9-tetradeoxy-l-glycero-l-manno-NulO), glycan analysis of strain UB4 indicated a 350-Da, possibly N-glycolyl Leg (3,5,7, 9-tetradeoxy-d-glycero-d-galacto-NulO) derivative with unknown C5,7 N-acyl moieties.80–82 Enzymes of both NulO pathways were produced as recombinant proteins and biochemically characterized, confirming their involvement in the biosynthesis pathway of CMP-Pse and CMP-Leg, respectively.79 Pse biosynthesis in ATCC 43037 essentially follows the UDP-sugar route described in Helicobacter pylori, while the pathway in strain FDC 92A2 corresponds to Leg biosynthesis in Campylobacter jejuni involving GDP-sugar intermediates.80–82 The modifications of either Ta. forsythia NulO were found to occur at the CMP-activated state.83
Compared to the wild-type strains, the NulO mutants exhibited significantly reduced biofilm formation on mucin-coated surfaces, suggestive of the involvement of these sugar acids in host-pathogen interactions or host survival.79,84 The occurrence of NulOs in bacteria is widespread and frequently linked to pathogenicity.85 In Ta. forsythia strains, several proposed virulence factors carry strainspecific modification, composed of either a Pse or a Leg derivative as the terminal sugar on an otherwise structurally identical protein-bound oligosaccharide. 79 A follow-up study shed light on the transfer of the NulO derivatives to a proximal N -acetylmannosaminuronic acid residue within the O -glycan structure,83 exemplified with the bacterium’s abundant S-layer glycoproteins. Bioinformatic analyses provided the candidate genes Tanf_01245 (strain ATCC 43037) and TFUB4_00887 (strain UB4), encoding a putative Pse and a Leg derivative transferase, respectively. These transferases have identical C-termini and contain motifs typical of glycosyltransferases (DXD) and bacterial sialyltransferases (D/ E -D/E-G and HP). They share homology to glycosyltransferase with a type B fold and TagB, an enzyme catalyzing glycerol transfer to an N -acetylmannosamine residue in teichoic acid biosynthesis.86 Cross-complementation of NulO deletion mutants with the nonnative NulO transferase was not successful, indicating high stringency of the enzymes. These Pse or Leg (derivative) transferases may serve as valuable tools for engineering of novel sialoglycoconjugates.6,83
Ta. forsythia exerts its pathogenic potential as a member of subgingival multispecies biofilms. The S-layer protein and its attached O-glycan were analyzed for their role in biofilm formation by employing an in vitro multispecies biofilm model mimicking the situation in the oral cavity.84 Different Ta. forsythia strains and mutants with characterized defects in cell surface composition were incorporated into the model, together with nine species of select oral bacteria.84,87 The influence of Ta. forsythia S-layer and attached glycan on the bacterial composition of the biofilms was analyzed quantitatively using colony-forming unit counts and quantitative real-time polymerase chain reaction, as well as qualitatively by fluorescence in situ hybridization and confocal laser scanning microscopy. This revealed that changes in the Ta. forsythia cell surface did not affect the quantitative composition of the multispecies consortium, with the exception of Campylobacter rectus cell numbers. The localization of Ta. forsythia within the bacterial agglomeration varied, depending on changes in the S-layer glycan, and this also affected its aggregation with Po. gingivalis. This suggests a selective role for the glycosylated Ta. forsythia S-layer in the positioning of this species within the biofilm, its co-localization with Po. gingivalis, and the prevalence of C. rectus. These findings might translate into a potential role for Ta. forsythia cell surface structures in the virulence of this species when interacting with host tissues and the immune system, from within or beyond the biofilm.84
Recently, Tannerella HOT-286 (phylotype BU063) isolate was identified as a novel filamentous Gram-negative anaerobic oral bacterium88 and subsequently grown in coculture with Propionibacterium acnes.89 Contrary to the related periodontal disease-associated pathobiont Ta. forsythia, it is considered a putative health-associated bacterium. As typical of Ta. forsythia,90 Tannerella HOT-286 could be grown in pure culture only if N-acetylmuramic acid (NAM) was provided in the media, although, surprisingly, this phenomenon is not likely to be due to deficiency in NAM synthesis genes. During further microbiological investigations it was shown that Tannerella HOT-286 possesses a prominent S-layer with a novel morphology [for comparison see Ref. 76], putatively made up of two proteins modified with an unknown glycan.89
3.2.2 Porphyromonas gingivalis
The Gram-negative anaerobic bacterium Po. gingivalis is another major pathogen in periodontal diseases. Based on previous results of lectin reactivity of Po. gingivalis,91 a recent study attempted to detect glycoproteins in lysates of Po. gingivalis cells by lectin blotting.92 Wheat germ agglutinin (WGA) affinity chromatography revealed the OmpA-like outer membrane protein of Po. gingivalis, designated Pgm6, as a major glycoprotein candidate, in addition to selected surface-associated gingipain proteases. 93 Pgm6 together with Pgm7 forms a heterotrimeric structure (Pgm6/7) that is responsible for the maintenance and stability of the outer membrane.92 Enriched Pgm6 was also positive with the ProQ-Emerald glycostain, reacted with an O-GlcNAc specific antibody, and could be deglycosylated with trifluoromethane sulfonic acid. Thus, it was concluded that OmpA-like proteins in Po. gingivalis carry O-GlcNAc moieties. Interestingly, as described for Ta. forsythia, Po. gingivalis has a functional type IX secretion system94 to which also potential glycoproteins could be targeted.
3.3
Other bacteria
3.3.1 Paenibacillus alvei
Pa. alvei CCM 2051T (Pa. alvei) is a Gram-positive, endospore forming, mesophilic bacterium that has gained attention as a secondary invader of diseased honeybee colonies infected with Melissococcus pluton, the causative agent of European foulbrood.95 The bacterium employs a protein O-glycosylation system that is so far exclusive to the bacterial domain, namely tyrosine O-glycosylation. This system equips the Pa. alvei S-layer protein SpaA with a branched polysaccharide consisting of, on average, 23 [→3)-β-d-Galp-1[α-d-Glcp-(1→6)]→4)-β-d-ManpNAc-(1→] repeating units linked via an adaptor with the structure-[GroA-2→OPO2→4-β-d-ManpNAc-(1→4)]→3)-αl-Rhap-(1→3)-α-l-Rhap-(1→3)-α-l-Rhap-(1→3)-β-d-Galp-(1→to specific tyrosine residues of SpaA96 (Fig. 2). Two predominant Tyr O-glycosylation sites are present on the S-layer protein, most probably corresponding to mono- and di-glycosylated S-layer protein visualized in periodic acid-Schiff (PAS)-stained sodium dodecyl sulfate polyacrylamide (SDS-PA) gels.97 A genomic 24.3-kb S-layer glycosylation (slg) gene cluster encodes the information necessary for the biosynthesis of this glycan chain within 18 open reading frames, working in concert with housekeeping functions of the bacterium.96
Pa. alvei CCM 2051T is the bacterium for which S-layer anchoring to the cell envelope via PGN-associated SCWP is currently best characterized.30 Binding is achieved by SLH domains, which are present in triplicate at the N-terminus of SpbA, but, generally, at the termini of S-layer and other extracellular proteins.25,27,98 The interaction of SLH domains with several SCWPs depends on a common, terminal ketal-pyruvate modification on an N-acetylmannosamine (ManNAc) residue of SCWPs that imparts a negative charge30,99; notably this modification is also present on the SCWP of Bacillus anthracis (Ba. anthracis), among others.100 SLH domains contain a highly conserved TRAE motif where the arginine residue has previously been shown to be critical to SCWP binding, suggesting that it interacts with the negatively charged pyruvate moiety of these SCWPs. SpaA possesses the conserved TRAE motif in its first SLH domain (SLH1) and variants of this motif, TVEE in its second (SLH2), and TRAQ in its third (SLH3) domain. These motifs were shown to contribute unequally to SCWP binding in Pa. alvei, where their mutation to TAAA resulted in 37%, 88%, and 50% of wild-type cell wall binding in pelleting assays, respectively.101 A comparison of the crystal structure of the SLH domain trimer from the Pa. alvei S-layer protein SpaA and the Ba. anthracis S-layer protein Sap revealed the three domains arranged in approximate threefold symmetry, where each SLH domain contributes one helix to a parallel three-helix bundle core and a second helix rotated ~ 90° from the core to form three lobes.29,30 The conserved TRAE motifs are located at the N-termini of the core helices adjacent to three grooves, suggesting that these grooves are the sites for SCWP binding. To define the molecular interaction basis between the SLH-domains and SCWP, crystals of the SLH domains of apo-SpaA, and in complex with synthesized, pyruvylated monosaccharide and disaccharide building blocks of Pa. alvei SCWP were analyzed. These structures revealed the contributions of many conserved residues to SCWP binding, notably including the most highly conserved residue SLH-Gly29.
This was confirmed by additional cocrystal structures, thermodynamic binding analysis, and microbiological functional assays for SLH-Gly29Ala mutants. SLH-Gly29 was shown to enable a peptide backbone flip essential for SCWP binding in both biophysical and cellular experiments. Furthermore, it was found that a significant domain movement mediates binding by two different sites in the SLH domain trimer, which may allow anchoring readjustment to relieve S-layer strain caused by cell growth and division.30
The functional coupling of ManNAc pyruvylation in SCWP and SLH-domain mediated anchoring of S-layer proteins is regarded as an ancestral mechanism of protein cell surface display in bacteria. In this context, the first functional proof of the CsaB enzyme catalyzing the widely unexplored pyruvylation reaction of ManNAc was provided102 using the Pa. alvei SCWP, consisting of pyruvylated disaccharide repeats [→4)-β-d-GlcNAc-(1→3)-4,6-Pyr-β-d-ManNAc-(1→]99 as a model structure. The underlying enzymatic pathway was reconstituted in vitro using synthesized acceptor compounds, and exploiting mass spectrometry (MS) and NMR spectroscopy for product characterization; it was found that CsaB-catalyzed pyruvylation of β-d-ManNAc occurs at the stage of the lipid-linked repeat.
3.3.2 Bacillus anthracis
In Ba. anthracis and Bacillus cereus (Ba. cereus), the two major S-layer proteins—the surface array protein Sap and extractable antigen 1 EA125—as well as the S-layerassociated proteins are retained by utilizing the interaction between pyruvylated ManNAc and SLH-domain,29 however providing a new facet of the mechanism. O-Acetylation of SCWPs of the Ba. cereus group of pathogens, which includes Ba. anthracis, is essential for the proper attachment of the S-layer proteins to their cell wall.103 Using a variety of pseudo-substrates and a chemically synthesized analogue of SCWP, PatB1 was identified as the SCWP O-acetyltransferase in Ba. cereus. Additionally, the crystal structure of PatB1 was reported, which provided detailed insights into the mechanism of this enzyme and defining a novel subfamily of the SGNH family of esterases and lipases. According to the proposed model, O-acetylation of SCWP requires the translocation of acetyl groups from a cytoplasmic source across the plasma membrane by PatA1 and PatA2 for their transfer to SCWP by PatB1.104
3.3.3 Kuenenia stuttgartiensis
Planctomycetes-Verrucomicrobia-Chlamydiae (PVC) bacteria have features that differentiate them from classical Gram-negative bacteria.105 The cell of the anammox organism K. stuttgartiensis, which belongs to the PVC bacteria, is surrounded by an S-layer representing the direct interaction zone of the bacterium with the environment. Previous studies with this model microbe suggested that the protein monomers of the S-layer Kustd1514 are glycosylated.106 In a recent study, the S-layer protein glycosylation was characterized in order to increase the knowledge of the cell surface characteristics of anammox bacteria. MS showed an O-glycan attached to 13 sites distributed over the entire 1591-amino acid S-layer protein. This glycan is composed of six monosaccharide residues, of which five are N-acetylhexosamine (HexNAc)
residues. Four of these HexNAc residues have been identified as N-acetylgalactosamine (GalNAc). The sixth monosaccharide of the glycan is a putative dimethylated deoxyhexose. Two of the HexNAc residues were also found to contain a methyl group, leading to an extensive degree of methylation of the glycan.107
3.3.4 Candidatus Brocadia
Recently, a high abundant glycoprotein, carrying a heterogeneous O-glycan structure, was identified by MS in the anammox granular sludge dominated by “Cand. Brocadia.” The potential glycosylation sequence motif was identical to that proposed for the S-layer protein of K. stuttgartiensis.107 The heavily glycosylated protein forms a large fraction of the extracellular polymeric substances and was also located by lectin staining. It is conceivable that glycoproteins play an important role in the structuring of anammox granules, comparable to the importance of glycans in the extracellular matrix of multicellular organisms.108
3.4 Archaea
During the past few years, considerable progress has been made in the structural and functional characterization of archaeal S-layer glycosylation (for review see Refs. 5, 21), involving mainly the organisms Haloferax volcanii (Hfx. volcanii), Sulfolobus acidocaldarius (Su. acidocaldarius), and Antarctic haloarchaea.109 Generally, archaeal S-layer proteins can play various roles in cellular processes, ranging from facilitating interactions between cells to maintaining cell stability.110
3.4.1 Haloferax volcanii
Recent work on the halophilic archaeon Hfx. volcanii extends the large body of research about S-layer protein glycosylation,21,111 deals with protein anchoring to the archaeal cell surface,110,112 and also covers more general aspects of the Hfx. volcanii life style.109 In the Hfx. volcanii, glycoproteins such as the S-layer glycoproteins can be modified by an N-linked pentasaccharide assembled by a series of Agl (archaeal glycosylation) proteins.52 Recently, straightforward analytical methods were applied to define the structure of Hfx. volcanii glycans that are attached to at least four of the seven putative N-glycosylation sites of the S-layer protein—Asn13, Asn83, Asn274, and Asn279. The N-glycans include a trisaccharide corresponding to glucuronic acid GlcA-(β1→4)-GlcA-(β1→4)-Glc-β1-Asn, a tetrasaccharide corresponding to methylO-4-GlcA-(β1→4)-GalA-(α1→4)-GlcA-(β1→4)-Glc-β1-Asn, and a pentasaccharide corresponding to hexose-1,2-[methyl-O-4-]GlcA-(β1→4)-GalA-(α1→4)-GlcA-β1,4Glc-β1-Asn (Fig. 2), in contrast to previous MS and radiolabeling experiments which had shown the hexose at the nonreducing end of the pentasaccharide to be mannose.113 The recent analysis also corrected an earlier assignment of the penultimate sugar as a methyl ester of a hexuronic acid, revealing this sugar to be a methylated GlcA. These new assignments are in agreement with what was already known of the Hfx. volcanii N-glycosylation pathway from previous genetic and biochemical efforts while providing new insight into this process.53
Across evolution, N-linked glycans are initially assembled on phosphorylated cytoplasmically oriented polyisoprenoids, with polyprenol (mainly C55-undecaprenol) fulfilling this role in bacteria and dolichol assuming this function in eukarya and archaea. The eukaryal and archaeal versions of dolichol can be distinguished on the basis of their length, degree of saturation, and by other traits. It is thought that the unusual physicochemical properties of their membrane lipids contribute to the abilities of archaea to withstand the physical challenges in their natural habitat.114,115 As in eukarya and bacteria, the archaeal cell is also surrounded by a phospholipid-based membrane. While eukaryal and bacterial phospholipids comprise fatty acid side chains linked to a 1,2-sn-glycerol-3-phosphate backbone via ester bonds, the archaeal counterparts are represented by isoprenoid hydrocarbon side chains linked to a 2,3-sn-glycerol-1-phosphate backbone via ether bonds. From Hfx. volcanii cells a series of C55 and C60 dolichol phosphates (Dol-P) were isolated, presenting saturated isoprene subunits at the α and ω positions. These are sequentially modified with the first, second, third, and methylated fourth sugar subunit comprising the first four subunits of the pentasaccharide N-linked to the S-layer glycoprotein.116 Moreover, when this glycan-charged phosphodolichol pool was examined in cells deleted of agl genes encoding the glycosyltransferases that participate in N-glycosylation by adding pentasaccharide residues one to four, the composition of the pool was correlated with S-layer protein N-glycan content in these mutants. In contrast, the fifth sugar of the pentasaccharide, identified as mannose in this study, is added to a different dolichol phosphate carrier. This represents the first evidence that in archaea, as in eukarya, the N-linked oligosaccharides are sequentially assembled from glycans originating from distinct phosphodolichol carriers.116 While information on the importance of N-glycosylation in eukaryotes and bacteria is available, the role of this posttranslational modification in archaea is not yet fully understood. Relying on Hfx. volcanii mutant strains, it was revealed that compromised N-glycosylation affected S-layer integrity and the transfer of a secreted reporter protein across the S-layer into the growth medium, as well as the conformation of the S-layer glycoprotein. Thus, by modifying N-glycosylation, Hfx. volcanii cells can change the way they interact with their surroundings.117
Rhomboids are scarcely known conserved intramembrane serine proteases involved in cell signaling processes and, typically, they are investigated in archaea.118,119
A rhomboid homologue deletion mutant (ΔrhoII) in Hfx. volcanii showed reduced motility, increased novobiocin sensitivity, and an N-glycosylation defect. Due to the mutation, many proteins showed variations in concentration, electrophoretic migration, or semi-tryptic cleavage. Potential RhoII targets include a PrsW protease homologue (which was less stable in the mutant), a predicted halocyanin, and six integral membrane proteins potentially related to the mutant glycosylation (SLG, Agl15) and cell adhesion/motility (flagellin1, HVO_1153, PilA1, and PibD) defects.119
Most prokaryote-secreted proteins are transported to the cell surface using general secretion (Sec) or twin-arginine translocation (TAT) pathway. The S-layer glycoprotein as the sole component of many archaeal cell walls was thought to be anchored to the cell surface by a C-terminal transmembrane segment.120 Recently, however, it was demonstrated that the Hfx. volcanii S-layer protein C-terminus is lipidated and subsequently removed in a process dependent on archaeosortase A (ArtA), a novel
peptidase. While the S-layer protein is a Sec substrate, in silico analyses revealed that of eight additional ArtA substrates predicted, two substrates also contain a predicted TAT signal peptide. These substrates include Hvo_0405 (probable cell surface fusion protein), which has a highly conserved tripartite structure that lies closer to the center of the protein than to its C-terminus, unlike other ArtA substrates identified to date. It could be shown, even given its atypical location, that this tripartite structure, which likely resulted from the fusion of genes encoding an ArtA substrate and a cytoplasmic protein, is processed in an ArtA-dependent manner. Using an Hvo_0405 mutant lacking the conserved “twin” arginines of the predicted TAT signal peptide, it was demonstrated that Hvo_0405 is indeed a TAT substrate and that ArtA substrates include both Sec and TAT substrates. These results demonstrated that both TAT and Sec substrates, which contain the conserved tripartite structure of predicted ArtA substrates, can be processed in an ArtA-dependent manner and that the tripartite structure need not lie near the C-terminus for this processing to occur.110
3.4.2 Sulfolobus acidocaldarius
In the thermoacidophilic archaeon Su. acidocaldarius, glycoproteins are modified by an N-linked tri-branched hexasaccharide with the structure {α-Man-(1→6)-} { α -Man-(1 → 4)-}-[(HO 3S → 6)-][ β -Glc-(1 → 4)-]- β -Qui-(1 → 3)- β -GlcNAc-(1 → 4)- βGlcNAc-(1→N-, 57 reminiscent of the N-glycans assembled in eukarya121 (Fig. 2). Previously, hexose-bearing Dol-P was detected in a Su. acidocaldarius Bligh-Dyer lipid extract. An improved protocol for extracting lipid-linked oligosaccharides allowed the detection of a dolichol pyrophosphate (Dol-PP) bearing the intact hexasaccharide, as well as its biosynthetic intermediates. Furthermore, evidence for N-glycosylation of two Su. acidocaldarius proteins by the same hexasaccharide and its derivatives was collected.122 Members of the genus Sulfolobus offer examples of archaeal N-glycosylation systems that combine both DolP- and DolPP-linked sugars and glycans. In both Su. acidocaldarius and Sulfolobus solfataricus, the N-linked glycan corresponds to a complex tri-branched oligosaccharide attached to target Asn residues via a di-GlcNAc core, reminiscent of the N-linked glycan decorating eukaryal glycoproteins.57
3.4.3 Antarctic haloarchaea
In the cold biosphere of Earth, archaea are prevalent and represented by diverse lineages. 123 Halohasta litchfieldiae represents ~ 44% and Halorubrum lacusprofundi ~ 10% of the hypersaline, perennially cold (≥ 20°C) Deep Lake community in Antarctica.109 Proteomics and microscopy were used to define physiological responses of these haloarchaea to growth at high (30°C) and low (10°C and 4°C) temperatures. Proteomic data indicated that both species respond to low temperature by modifying their cell envelope by including protein N-glycosylation, maintaining osmotic balance and translation initiation, and modifying RNA (ribonucleic acid) turnover and tRNA (transfer RNA) modification. The protective S-layer of haloarchaea is composed of glycoproteins; Hht. litchfieldiae encodes one S-layer protein (halTADL_1043) and Hrr. lacusprofundi encodes two S-layer proteins (Hlac_0412, Hlac_2976).124 According
to these recent data, growth conditions could play a role in regulating S-layer protein expression and N-glycosylation is required for their assembly and function. In both species, a glycosyltransferase (halTADL_1783; Hlac_0164) homologous to Hfx. volcanii protein HVO_1613 (encoding a dolichyl-phosphate hexose transferase) had higher abundance at low temperature. Proteomic data indicated that S-layer glycosylation is regulated by growth temperature in specific ways for Hht. litchfieldiae and Hrr. lacusprofundi. Sulfation of S-layer glycans in Hrr. lacusprofundi may also be growth temperature regulated.
Thus, Hht. litchfieldiae and Hrr. lacusprofundi share a number of strategies for growing under conditions that approximate their natural environment (low temperature and high salt). However, this is an area that requires further investigation, as limited knowledge exists as to how alteration of N-glycosylation enables cells to adapt to different environmental conditions.109
4 Flagella/Archaella and fimbriae
Flagellins are abundant bacterial proteins comprising the flagellar filaments that propel bacterial movement.125 Some species express multiple flagellins, in contrast to model systems such as Escherichia coli that possess only one flagellin protein.126 Flagellin glycosylation has been observed on an increasing number of Gram-negative and Gram-positive bacteria. Many of these are pathogens that exhibit enormous differences in the strain-specific glycan structures; glycosylation of flagellar proteins is considered a major virulence factor [for review see Ref. (5)].
Fimbriae, also referred to as “attachment pili,” are typically thinner and shorter than a flagellum and are present in high numbers at the cell surface. Fimbriae are one of the primary mechanisms of virulence of, for instance, E. coli, Staphylococcus sp. and Streptococcus sp., because their presence greatly enhances these organisms’ ability to attach to the host and cause disease.127 Yet, information about fimbrial glycosylation is scarce. In both bacteria and archaea, the biosynthesis of pilus-related structures involves a set of core components.128–130
4.1 Bacteria
4.1.1 Paenibacillus alvei
The basis of flagellin glycosylation has been studied for various Gram-negative bacteria, but less is known about flagellin glycans of Gram-positive bacteria including Pa. alvei CCM 2051T.131 The microbe swarms vigorously on solidified culture medium, with swarming relying on functional flagella as evidenced by abolished biofilm formation of a nonmotile Pa. alvei mutant defective in the flagellin protein Hag (PAV_2c01710). Analysis of purified flagellin demonstrated that the 30-kDa Hag protein is modified with an O-linked trisaccharide comprising one hexose and two HexNAc residues, at three glycosylation sites. Downstream of the hag gene on the bacterial chromosome, two open reading frames (PAV_2c01630, PAV_2c01640)
encoding putative glycosyltransferases were shown to constitute a flagellin glycosylation island. Mutants defective in these genes faced loss of extracellular flagella production and bacterial motility. Thus, flagellin glycosylation in Pa. alvei is pivotal to flagella formation and bacterial motility in vivo.131 Flagellin glycosylation constitutes a second protein O-glycosylation system in this bacterium, in addition to the wellinvestigated S-layer tyrosine O-glycosylation pathway.5,131
4.1.2 Selenomonas sputigena
Recently, in the Gram-negative, anaerobic, crescent-shaped bacterium Se. sputigena, the first glycosylated flagellin was reported.132 Selenomonas are colonizers of the digestive system, where they act at the interface between health and disease. Se. sputigena is considered a potential human periodontal pathogen, but information on its virulence factors and underlying pathogenicity mechanisms is scarce. This microbe produces a diversely and heavily O-glycosylated flagellin C9LY14 as a major cellular protein, which carries various hitherto undescribed rhamnose- and GlcNAc-linked Oglycans in the range from mono- to hexasaccharides. A comprehensive MS analysis revealed extensive glycan macro- and microheterogeneity identified from 22 unique glycopeptide species. From the multiple sites of glycosylation, five were unambiguously identified on the 437-amino acid C9LY14 protein (Thr149, Ser182, Thr199, Thr259, and Ser334). The complex O-glycans showed additional modifications by methylation and putative acetylation. Some O-glycans carried hitherto undescribed residues/modifications based on their respective m/z values, reflecting the high diversity of native Se. sputigena flagellin. In fact, the Se. sputigena flagellin C9LY14 is one of the most heavily O-glycosylated flagellins described to date.132
4.1.3 Porphyromonas gingivalis
The periodontal pathogen Po. gingivalis67 targets through its (minor) fimbria glycoprotein Mfa1 the C-type lectin receptor DC-SIGN to support invasion and persistence within human monocyte-derived dendritic cells (DCs). The DCs respond by inducing an immunosuppressive and Th2-biased CD4+ T-cell response. In previous experiments, DC-SIGN ligation could be abrogated by using DC-SIGN-blocking agents or agonists, including fucose (Fuc), mannose (Man), and mannan.133 The predicted molecular mass for Mfa1 is approximately 61 kDa, but Mfa1 from the native minor fimbriae migrated as a ProQ Emerald glycostain-positive band at 67 kDa, which might be an indication of a PTM.133 In a recent study, the native Mfa1 protein was purified by ion-exchange chromatography followed by sequencing by tandem mass spectrometry (MS/MS), confirming its identity and revealing two putative N-glycosylation motifs as well as numerous putative O-glycosylation sites.134 Monosaccharide compositional analysis by gas chromatography–mass spectrometry (GC–MS) confirmed that the minor fimbrial protein contains the DC-SIGN targeting carbohydrates Fuc, Man, GlcNAc, and N-acetylgalactosamine (GalNAc) in an approximate molar ratio of 2.0/4.0/3.5/1.0 (with GalNAc set to 1.0); additionally, xylose, Gal, and Glc were found (7.0/9.0/22.0, referenced to GalNAc). Glycosylation could be partially removed by treatment with β1,4-galactosidase, but not by classic N- and O-linked deglycosidases.
Overall, these results indicate that the minor fimbria, forming fibers of approximately 200 nm in length, are glycosylated with DC-SIGN-binding motifs that likely account for the reported ability of Po. gingivalis to bind to and invade DCs, resulting in an immunosuppressive DC response.
4.2 Archaea
4.2.1 Haloferax volcanii
N-Glycosylation is not only important for archaella assembly but is obviously also involved in pilus formation in Hfx. volcanii.135 It was found that of six Hfx. volcanii adhesion pilins, PilA1 and PilA2, the most abundant pilins in pili of wild-type and ΔaglB strains, are modified under planktonic conditions in an AglB-dependent manner by the same pentasaccharide as detected on Hfx. volcanii archaella. However, unlike wildtype cells, which have surfaces decorated with discrete pili and form a dispersed layer of cells on a plastic surface, ΔaglB cells have thick pili bundles and form microcolonies. Growth of wild-type cells in low salt media, a condition that decreases AglB glycosylation, also stimulates microcolony formation and inhibits motility, supporting the hypothesis that N-glycosylation plays an important role in regulating the transition between planktonic to sessile cell states as a response to stress.5,135
5 Adhesins, bacteriocins, invasins, and glycocins
Bacteria use various strategies to compete in their ecological niche; most bacterial glycoproteins identified to date are virulence factors of pathogenic bacteria, such as adhesins, invasins, bacteriocins,136 and glycocins (glycosylated bacteriocins).
Bacteriocins are ribosomally synthesized, posttranslationally modified antibacterial peptides (RiPPs)137 and it has been postulated that the majority of Gram-positive bacteria produce one or more of these natural products. They are used in food preservation and also considered as potential alternatives to antibiotics.138 The majority of bacteriocins from Gram-positive bacteria were traditionally divided into two major classes, namely lantibiotics, which are posttranslationally modified bacteriocins and unmodified bacteriocins. The last decade has seen an expanding number of RiPPs in Grampositive bacteria that have antibacterial activity. In addition, the three-dimensional (3D) structures of a number of modified and unmodified bacteriocins have been elucidated with a focus on the chemical and 3D structures of these peptides, and their interactions with receptors and membranes, structure–function relationships, and possible modes of action.136
5.1 Streptococcus gordonii
Many pathogenic bacteria, including Sc. gordonii, possess a pathway for the cellular export of a single serine-rich repeat (SRR) protein that mediates the adhesion of bacteria to host cells and the extracellular matrix.139 In general, Gram-positive
bacterial genomes have a unique SRR glycoprotein-encoding gene. The Sc. gordonii adhesin GspB is sequentially O-glycosylated by three cytosolic enzymes (GtfA/B, Nss, and Gly) which attach GlcNAc and Glc residues to Ser/Thr sites.140 Modified GspB is transferred from the last glycosyltransferase to the Asp1/2/3 complex of accessory proteins.141 Crystal structures revealed that both Asp1 and Asp3 are related to carbohydrate-binding proteins, suggesting that they interact with carbohydrates and bind glycosylated adhesin. It was further observed that Asp1 also has an affinity for phospholipids, which is attenuated by Asp2. This supports a model in which Asp1 mediates the interaction of the Asp1/2/3 complex with the lipid bilayer for targeting the mature GspB glycoprotein to the export machinery.141,142
Sc. gordonii and Streptococcus sanguinis are typically found among the normal oral microbiota but can also cause infective endocarditis. The SRR adhesins of these organisms contain “Siglec-like” binding regions (SLBRs) that mediate attachment to α2,3linked sialic acids on human glycoproteins.143 Two known receptors for the Siglec-like adhesins are the salivary mucin MG2/MUC7 and platelet GPIbα, and the interaction of streptococci with these targets may contribute to oral colonization and endocarditis, respectively. Four recently investigated SLBRs recognize a surprisingly small subset of plasma proteins that are extensively O-glycosylated. The preferred plasma protein ligands for a sialyl-T antigen-selective SLBR are proteoglycan 4 (lubricin) and inter-α-trypsin inhibitor heavy chain H4. Conversely, the preferred ligand for a 3′-sialyllactosamineselective SLBR is glycocalicin (the extracellular portion of platelet GPIbα). All four investigated SLBRs recognize the complement factor 1 inhibitor but detect distinctly different glycoforms of this key regulator of the complement and kallikrein protease cascades. The four plasma ligands have potential roles in thrombosis and inflammation, and each has been cited as a biomarker for one or more vascular or other diseases.143
5.2 Streptococcus salivarius
The pioneer colonizer and commensal bacterium of the human gastrointestinal tract, Sc. salivarius, has strong adhesive properties but the molecular determinants of this adhesion have remained uncharacterized.144 It was demonstrated that Sc. salivarius expresses three large and glycosylated surface exposed proteins—SrpA, SrpB, and SrpC—that show characteristics of SRR glycoproteins and are secreted through the accessory SecA2/Y2 system. Two glycosyltransferases—GtfE/F—unusually encoded outside of the secA2/Y2 locus perform the first step of the sequential glycosylation process, which is crucial for SRR activity. SrpB and SrpC play complementary adhesive roles involved in several steps of the colonization process: autoaggregation, biofilm formation, and adhesion to a variety of host epithelial cells and components. SrpA, SrpB, and SrpC are the main factors underlying the multifaceted adhesion of Sc. salivarius and, therefore, play a major role in host colonization.144
5.3 Streptococcus agalactiae
Streptococcosis caused by Sc. agalactiae is one of the most important diseases in the tilapia fish aquaculture industry.145 To gain insight into how the microbe adheres to
the intestinal epithelium of tilapia (Oreochromis sp.), recently, the role of its capsule during adhesion was evaluated in an ex vivo infection model. Wild-type strain Sc. agalactiae SaTiBe08-18 recovered from tilapia had reduced adhesion (P < 0.0001) in comparison with its unencapsulated mutant SaTiBe08-18 Δcps. When the wild-type strain was treated with sterile saline solution (pH 5) before infection of intestine explants, the adhesion was reached. This suggests that the capsule impairs the adhesion of Sc. agalactiae to tilapia intestine and that the acidic milieu could regulate adherence of encapsulated strains. Interestingly, GlcNAc was found on the surface of adherent Δcps but not on the capsule in the wild-type strain. This difference could be explained by the exposure of GlcNAc which is part of PGN-anchored Lancefield group B antigen in group B Streptococcus (GBS),146 to which Sc. agalactiae is affiliated, and also may be related with better exposure of glycosylated adhesins in unencapsulated fish GBS. Understanding capsular regulation during adhesion of Sc. agalactiae may provide new leads to find a successful anti-adherence therapy to prevent streptococcosis in tilapia.145
5.4 Streptococcus mutans
In Sc. mutans the expression of the collagen- and laminin-binding surface glycoprotein Cnm mediates binding to extracellular matrix proteins, endothelial cell invasion, and virulence in the Galleria mellonella invertebrate model.147 There are indications that Cnm undergoes O-glycosylation with GlcNAc residues within a threonine-rich domain, catalyzed by the glycosyltransferase PgfS.148 To further characterize Cnm as a virulence factor, the cnm gene from Sc. mutans strain OMZ175 was expressed in the nonpathogenic Lactococcus lactis NZ9800 (Lc. lactis) using a nisin-inducible system.149 Despite the absence of the machinery necessary for Cnm glycosylation, Western blot and immunofluorescence microscopy analyses demonstrated that Cnm was effectively expressed and translocated to the cell wall of Lc. lactis. Similar to Sc. mutans, expression of Cnm in Lc. lactis enabled robust binding to collagen and laminin, invasion of human coronary artery endothelial cells, and increased virulence in G. mellonella. Using an ex vivo human heart tissue colonization model, it was shown that Cnm-positive strains of either Sc. mutans or Lc. lactis outcompete their Cnm-negative counterparts for tissue colonization. Finally, Cnm expression facilitated Lc. lactis adhesion and colonization in a rabbit model of infective endocarditis.147
5.5 Staphylococcus aureus
St. aureus is a major human pathogen that causes life-threatening infections due to its ability to attach to surfaces, form biofilms, and persist inside the host.150 Only recently the impact of protein glycosylation in St. aureus has been unraveled. One of previously identified virulence factors in St. aureus pathogenesis is plasmin-sensitive surface protein (Pls). In two different strains of St. aureus two and four Pls, respectively, were now shown to be glycosylated. The pls gene is encoded by the staphylococcal cassette chromosome (SCC) mec type I in MRSA (methicillin-resistant St. aureus) that also encodes the methicillin resistance-conferring mecA and further genes. Downstream