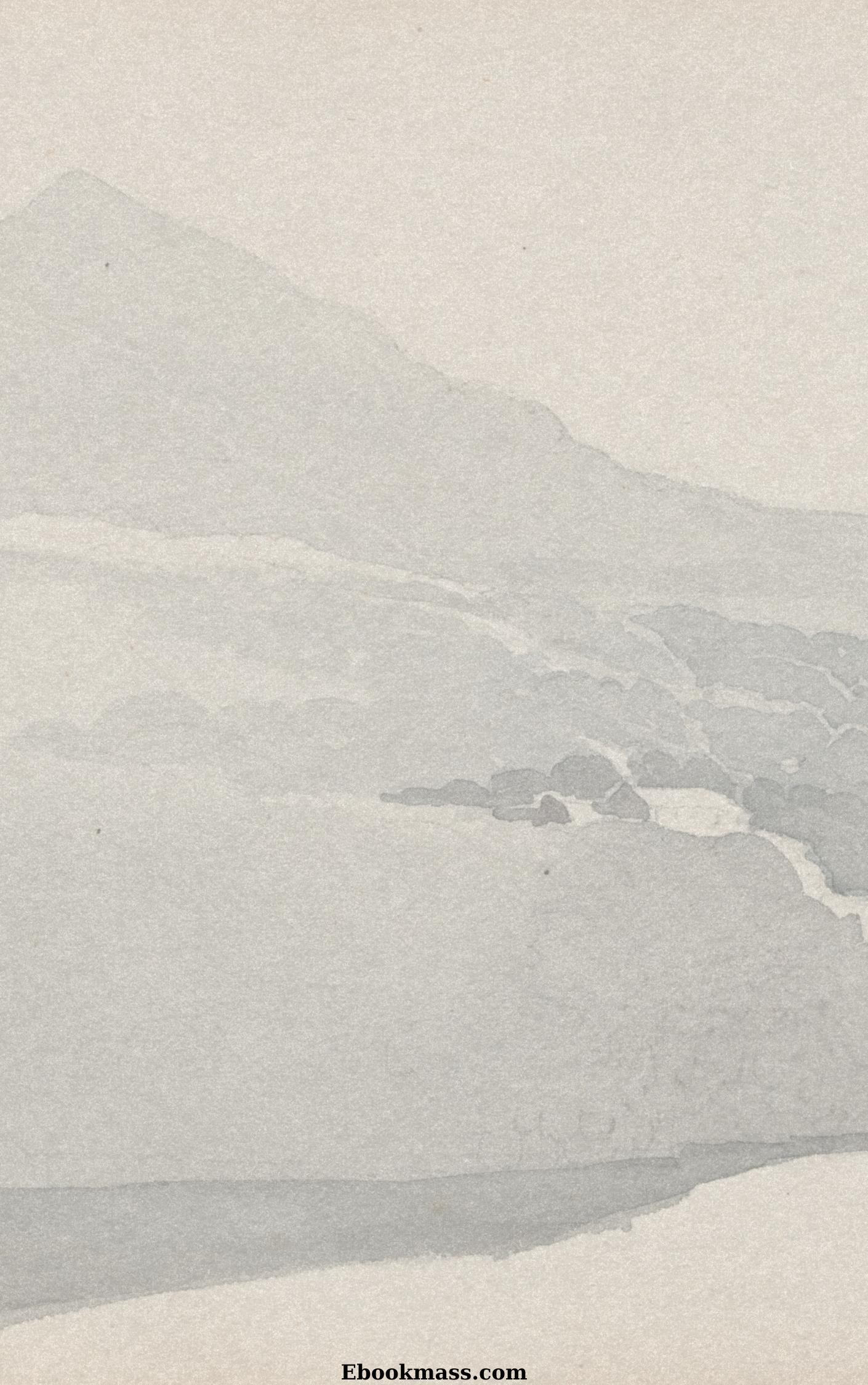
Novelstrategiesfortheformulationand processingofaluminummetal-organicframeworkbasedsensingsystemstowardenvironmental monitoringofmetalionsYongbiaoHua
https://ebookmass.com/product/novel-strategies-for-theformulation-and-processing-of-aluminum-metal-organicframework-based-sensing-systems-toward-environmentalmonitoring-of-metal-ions-yongbiao-hua/
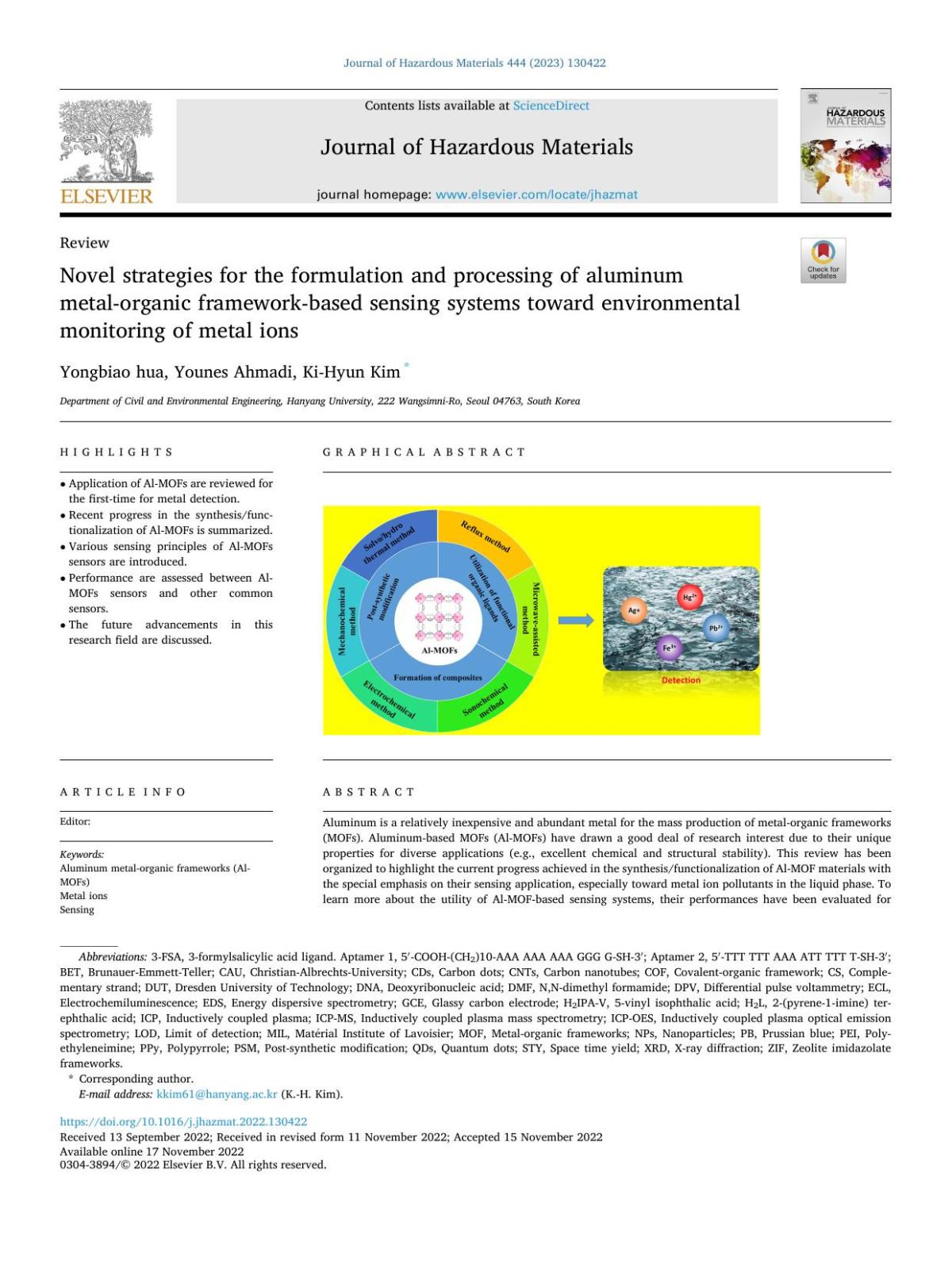
Instant digital products (PDF, ePub, MOBI) ready for you
Download now and discover formats that fit your needs...
Metal-Organic Frameworks (MOFs) for Environmental Applications Sujit K. Ghosh
https://ebookmass.com/product/metal-organic-frameworks-mofs-forenvironmental-applications-sujit-k-ghosh/
ebookmass.com
Rare Earth Metal-Organic Framework Hybrid Materials for Luminescence Responsive Chemical Sensors Bing Yan
https://ebookmass.com/product/rare-earth-metal-organic-frameworkhybrid-materials-for-luminescence-responsive-chemical-sensors-bingyan/
ebookmass.com
Metal-free synthetic organic dyes Kruger
https://ebookmass.com/product/metal-free-synthetic-organic-dyeskruger/
ebookmass.com
Replacing the Dead: The Politics of Reproduction in the Postwar Soviet Union Mie Nakachi
https://ebookmass.com/product/replacing-the-dead-the-politics-ofreproduction-in-the-postwar-soviet-union-mie-nakachi/
ebookmass.com
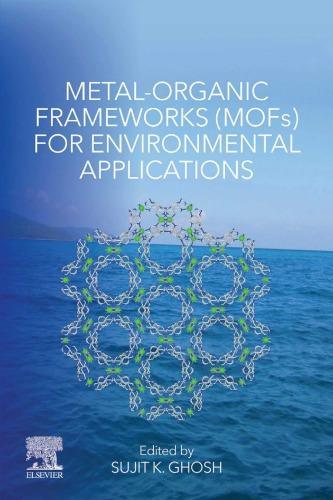
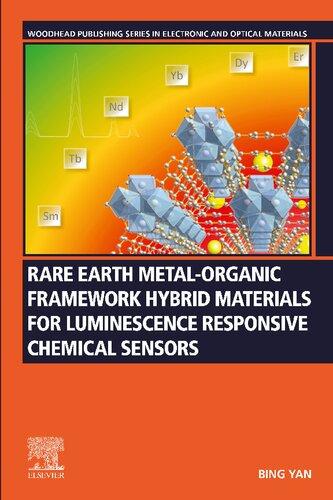
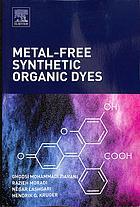
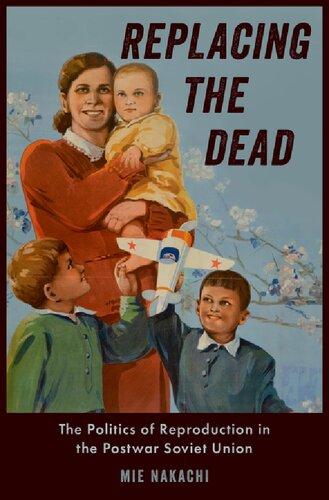
https://ebookmass.com/product/bad-trust-michael-a-kahn/
ebookmass.com
Consuming identities : visual culture in nineteenthcentury San Francisco 1st Edition Lippert
https://ebookmass.com/product/consuming-identities-visual-culture-innineteenth-century-san-francisco-1st-edition-lippert/
ebookmass.com
Zero-Carbon Industry: Transformative Technologies and Policies to Achieve Sustainable Prosperity Jeffrey Rissman
https://ebookmass.com/product/zero-carbon-industry-transformativetechnologies-and-policies-to-achieve-sustainable-prosperity-jeffreyrissman/
ebookmass.com
The Aces' Sons Books 1-3: Limited Edition Box Set Nicole Jacquelyn
https://ebookmass.com/product/the-aces-sons-books-1-3-limited-editionbox-set-nicole-jacquelyn/
ebookmass.com
Total manufacturing assurance : controlling product quality, reliability, and safety 2nd Edition John Cesarone
https://ebookmass.com/product/total-manufacturing-assurancecontrolling-product-quality-reliability-and-safety-2nd-edition-johncesarone/
ebookmass.com
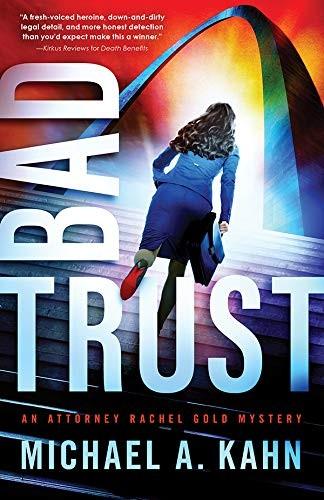
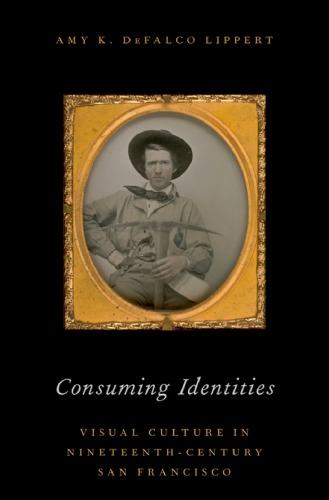
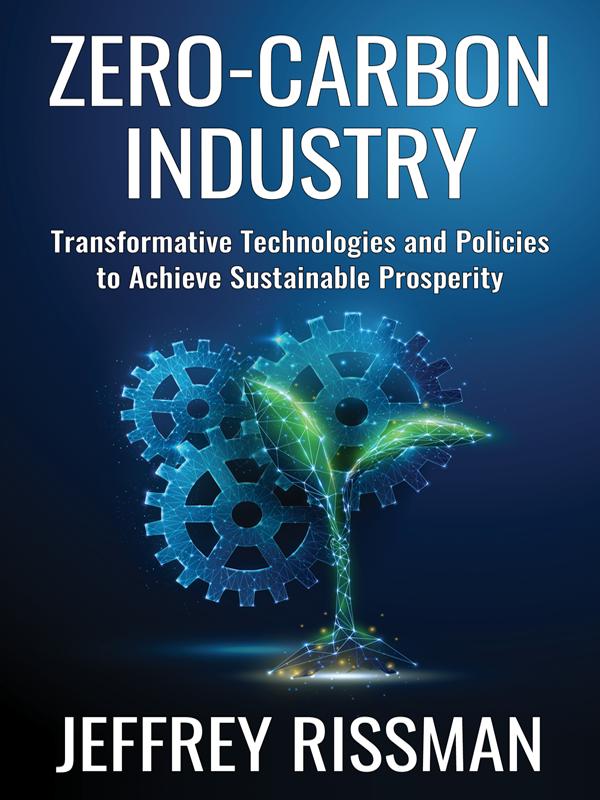
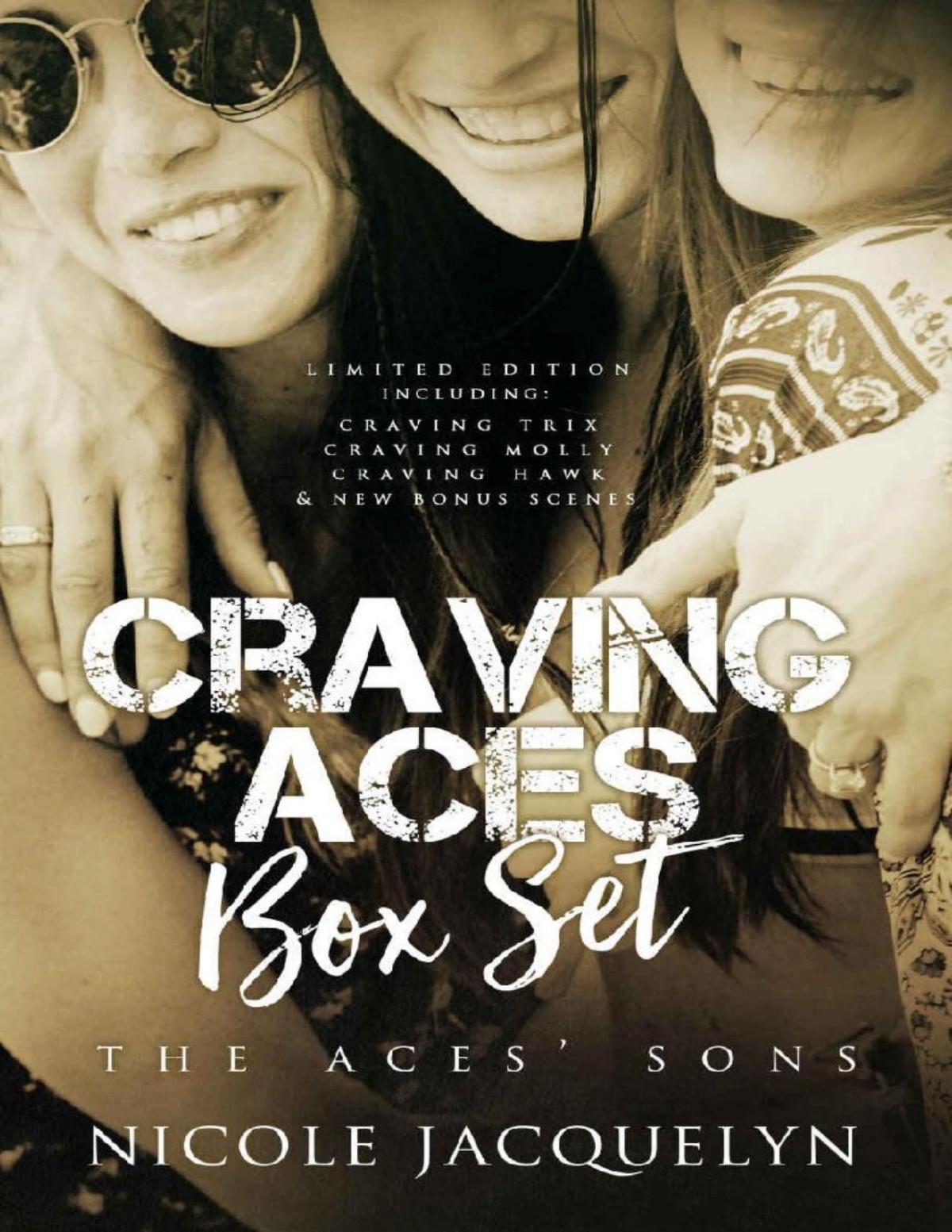
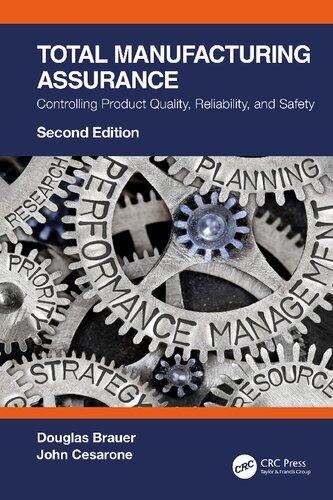
Mcnaughton
https://ebookmass.com/product/samuel-beckett-and-the-politics-ofaftermath-james-mcnaughton/
ebookmass.com
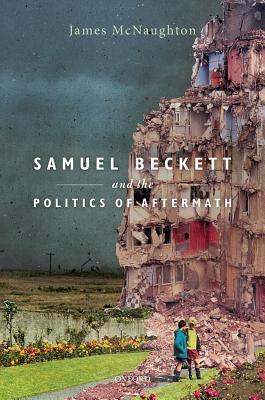
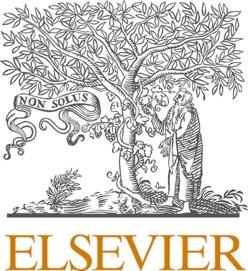
Review
Contents lists available at ScienceDirect
Journal of Hazardous Materials
journal homepage: www.elsevier.com/locate/jhazmat
Novel strategies for the formulation and processing of aluminum metal-organic framework-based sensing systems toward environmental monitoring of metal ions
Yongbiao
hua, Younes Ahmadi, Ki-Hyun Kim *
Department of Civil and Environmental Engineering, Hanyang University, 222 Wangsimni-Ro, Seoul 04763, South Korea
HIGHLIGHTS
• Application of Al-MOFs are reviewed for the first-time for metal detection.
• Recent progress in the synthesis/functionalization of Al-MOFs is summarized.
• Various sensing principles of Al-MOFs sensors are introduced.
• Performance are assessed between AlMOFs sensors and other common sensors.
• The future advancements in this research field are discussed.
GRAPHICAL ABSTRACT
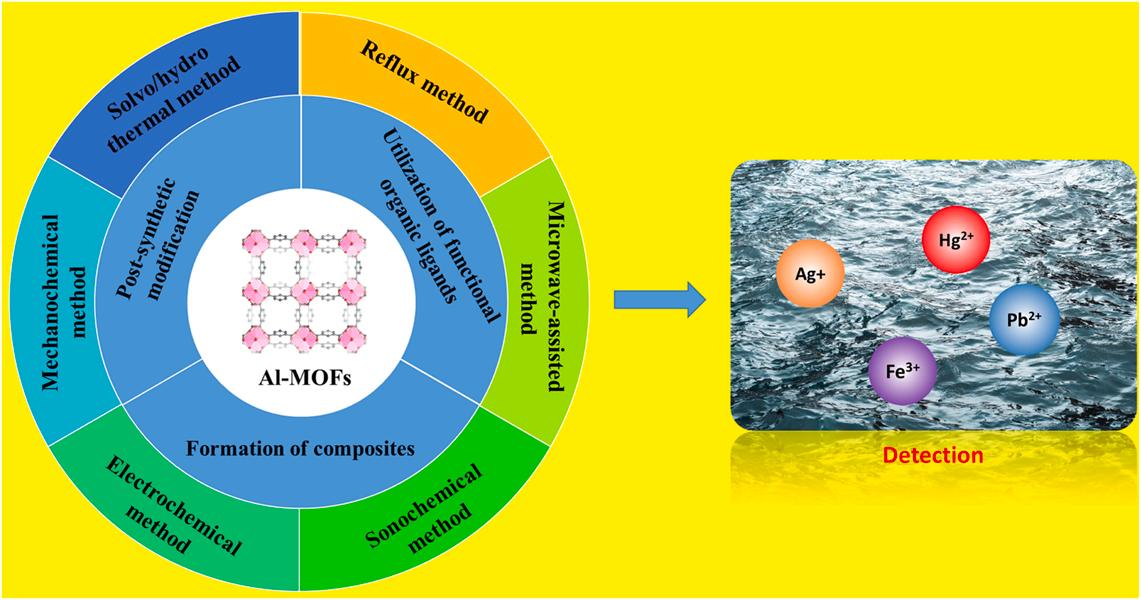
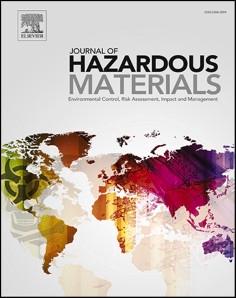
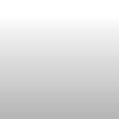
ARTICLE INFO
Editor:
Keywords:
Aluminum metal-organic frameworks (AlMOFs)
Metal ions
Sensing
ABSTRACT
Aluminum is a relatively inexpensive and abundant metal for the mass production of metal-organic frameworks (MOFs). Aluminum-based MOFs (Al-MOFs) have drawn a good deal of research interest due to their unique properties for diverse applications (e.g., excellent chemical and structural stability). This review has been organized to highlight the current progress achieved in the synthesis/functionalization of Al-MOF materials with the special emphasis on their sensing application, especially toward metal ion pollutants in the liquid phase. To learn more about the utility of Al-MOF-based sensing systems, their performances have been evaluated for
Abbreviations: 3-FSA, 3-formylsalicylic acid ligand. Aptamer 1, 5′ -COOH-(CH2)10-AAA AAA AAA GGG G-SH-3′ ; Aptamer 2, 5′ -TTT TTT AAA ATT TTT T-SH-3′ ; BET, Brunauer-Emmett-Teller; CAU, Christian-Albrechts-University; CDs, Carbon dots; CNTs, Carbon nanotubes; COF, Covalent-organic framework; CS, Complementary strand; DUT, Dresden University of Technology; DNA, Deoxyribonucleic acid; DMF, N,N-dimethyl formamide; DPV, Differential pulse voltammetry; ECL, Electrochemiluminescence; EDS, Energy dispersive spectrometry; GCE, Glassy carbon electrode; H2IPA-V, 5-vinyl isophthalic acid; H2L, 2-(pyrene-1-imine) terephthalic acid; ICP, Inductively coupled plasma; ICP-MS, Inductively coupled plasma mass spectrometry; ICP-OES, Inductively coupled plasma optical emission spectrometry; LOD, Limit of detection; MIL, Mat´ erial Institute of Lavoisier; MOF, Metal-organic frameworks; NPs, Nanoparticles; PB, Prussian blue; PEI, Polyethyleneimine; PPy, Polypyrrole; PSM, Post-synthetic modification; QDs, Quantum dots; STY, Space time yield; XRD, X-ray diffraction; ZIF, Zeolite imidazolate frameworks.
* Corresponding author.
E-mail address: kkim61@hanyang.ac.kr (K.-H. Kim).
https://doi.org/10.1016/j.jhazmat.2022.130422
Received 13 September 2022; Received in revised form 11 November 2022; Accepted 15 November 2022
Availableonline17November2022 0304-3894/©2022ElsevierB.V.Allrightsreserved.
Performance
Post-synthetic modification
Nano-particles
1. Introduction
diverse metallic components in reference to many other types of sensing systems (in terms of the key quality assurance (QA) criteria such as limit of detection (LOD)). Finally, the challenges and outlook for Al-MOF-based sensing systems are discussed to help expand their real-world applications.
To date, numerous types of sensing systems have been developed for various objectives. For instance, humans can avoid the consumption of toxins using the olfactory system (which provides the sense of smell), as it allows to differentiate between fresh and expired food (Tricoli et al., 2010). Such natural sensors have inspired the scientific community to design and construct devices that could rapidly determine the quantity (and/or quality) of the target components in the surrounding environment. As a result, various sensing systems (e.g., chemical and biological sensors) are now available in diverse fields such as environmental monitoring, food safety, and agriculture applications (Schroeder et al., 2018; Banica, 2012).
The presence of emerging environmental pollutants (such as metal ions) poses a significant threat to human health (e.g., endocrine disruption, congenital disorder, and carcinogenesis) (Pereira et al., 2015; Ramírez-Malule et al., 2020; Wilkinson et al., 2017; Lodeiro et al., 2019; Ahmed et al., 2021). The application of chemical sensing technology is considered a promising approach towards the rapid detection of toxic metal ions in bodies of water. Such technology is growing due to its practicality, especially wirh respect to high sensitivity (i.e., detection of targets even at low concentrations) and facile operation. Generally, chemical sensors are composed of sensing and transduction units to detect and translate the signals into easily readable electrical and/or optical signals (Paixao and Reddy, 2017). The selection and design of the sensing materials employed in sensor platforms are thus important to obtain enhanced sensing performance (e.g., sensitivity and stability) (Fang et al., 2018; Paolesse et al., 2017).
In the construction of an ideal sensing system, the properties of sensing materials are important for obtaining their optimum performance (e.g., in terms of highly exposed surface, numerous active sites available for the analytes to bind/react, good mechanical properties, and device flexibility) (Majhi et al., 2022). In this context, metal-organic frameworks (MOFs) have drawn great attention for sensing applications due to their advantageous properties (e.g., large surface areas, cavity structures, and tunable porosity) (Fang et al., 2018; Kreno et al., 2012). MOFs are porous coordination polymers formed by the coordination of metal ions/clusters and organic bridging linkers/ligands (Fig. 1) (Zhou et al., 2012). MOFs with desired properties (e.g., porous nature and crystalline) can be constructed by adjusting their components (e.g., various metal ions and organic ligands) and/or their preparation procedures (and activation methods) (Lu et al., 2022). Indeed, such flexibilities effectively endow MOFs with great potential advantages for sensing applications. In addition, the reversibility of MOFs for the adsorption/desorption of analytes can further ensure their recyclability (Li et al., 2020a; Liu et al., 2014). The rigid structure of MOFs is helpful in predicting the formation of interactions between analytes and the MOF surface at the atomic/molecular level (Li et al., 2020a; Liu et al., 2014). Despite the many advantages of MOFs, many of them also suffer
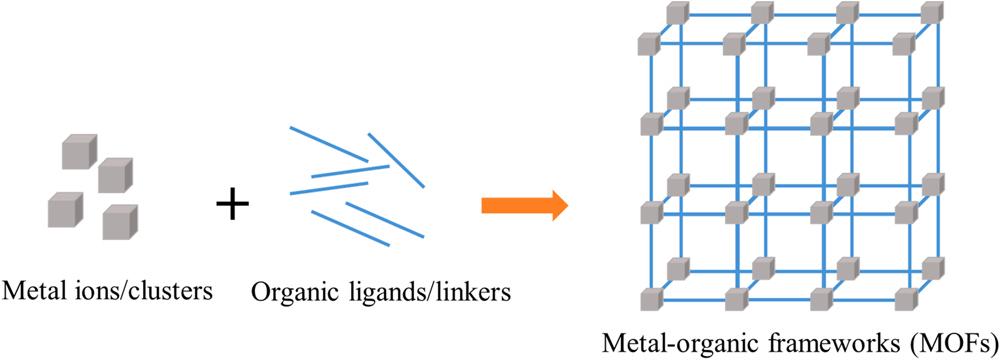
from low endurance in harsh environments (e.g., high temperature and moisture) (Ding et al., 2019; Yuan et al., 2018). For example, some MOFs synthesized by divalent cations (e.g., Zn2+ , Cu2+ , and Co2+) are often reported to have several disadvantages in terms of chemical and/or hydrothermal instabilities (Devic and Serre, 2014).
To scale up the production of MOFs for diverse applications, it is important to develop new strategies for their fabrication such as the use of highly abundant, low cost, lightweight, and high valence metals (Devic and Serre, 2014; Wu et al., 2020). Among a plethora of metal species (e.g., zirconium, iron, and chromium) present on the earth, aluminum is one of the most abundant metallic components in the earth crust (8.3% by weight) (McLeod and Shaulis, 2018). Moreover, due to the low toxicity and low cost, the selection of highly charged metal cationic aluminum (i.e., Al3+) can be favorable in the synthesis of a stable and robust MOF through the build-up of strong metal-ligand bonds (Devic and Serre, 2014; Wu et al., 2020; Schilling et al., 2016). Further, Al-MOFs can also take the advantage of a green solvent (e.g., water) unlike other MOFs (e.g., zirconium MOFs) generally prepared with toxic solvents (e.g., DMF) (Schilling et al., 2016; Chen et al., 2019a). As such, the ton-scale production of Al-MOFs can be achieved in a water-based route (Gaab et al., 2012). The utility of Al-MOFs is hence recognized from various fields (such as gas storage, sensing, adsorption, separations, and catalysis) with the aid of such meritful properties (Shi et al., 2021a; Sikka et al., 2022; Kim et al., 2019; Fan et al., 2019; Wang et al., 2020a; Loiseau et al., 2015) (Fig. 2). Moreover, the good water stability and high capacity of Al-MOFs for adsorption in aqueous system was also beneficial toward the sensing application such as metal ions in aqueous system (Samokhvalov, 2018). Al-MOFs can easily be constructed with stimuli responsive organic ligands (e.g., 2-aminoterephthalic acid) with specific optical characteristics (e.g., luminescence) for sensing application (Lin et al., 2020). As such, Al-MOFs chemosensors have been developed for the detection of various pollutants (e.g., pharmaceuticals) (Li et al., 2019; Zhang et al., 2019a). For instance, the NH2-MIL-53 (Al) has been utilized for fluorescent detection of pharmaceuticals (e.g., tetracyclines) in liquid milk (Li et al., 2019). The NH2-MIL-53 (Al) nanosensor exhibited specific recognition toward tetracyclines through the formation of hydrogen-bonding interactions between the -NH2 of the NH2-MIL-53 (Al) and the –OH of tetracyclines. As
Fig. 2. Growth in the annual number of publications on Al-MOFs and their applications in sensing metal ions(Sources: Web of Science, Google Scholar, and Scopus databases) based on several key words(e.g., aluminum metal-organic frameworks, metal ions, sensing, and Al-MOFs).
such, the NH2-MIL-53 (Al) nanosensor was demonstrated to quantify tetracyclines by inducing the fluorescent quenching (e.g., transferring the electrons of –NH2/-COOH from NH2-MIL-53 (Al) to the –CO/-OH) (Li et al., 2019).
To date, enormous scientific efforts have been devoted to the synthesis and application (e.g., sorption, catalysis, and membrane) of AlMOFs (Wu et al., 2020; Gaab et al., 2012; Loiseau et al., 2015; Samokhvalov, 2018; Stock, 2014; Steenhaut et al., 2021; Aguirre-Díaz et al., 2017). For instance, Samokhvalov introduced the application of Al-MOFs as advanced sorbent for the removal of various environmental pollutants (Samokhvalov, 2018). The utilization of Al-MOFs in membrane techniques has also been dealt in various fields (e.g., gas separation and pervaporation) (Wu et al., 2020). Nonetheless, the application of Al-MOFs for sensing applications toward metal ions has scantily been reported. As a result, we seek to provide a comprehensive overview on sensing application of Al-MOFs against various metal ion pollutants in the aqueous system in relation to their synthesized strategies. For this purpose, we focused on the significance of experimental variables in various methods employed for the synthesis of Al-MOFs (e.g., solvo/hydrothermal, sonochemical, and microwave-assisted methods). Further, the merits and disadvantages of such strategies were addressed for improving their preparation as well as sensing applications. Up-to-date information on the approaches available for the functionalization of Al-MOFs is also provided to help expand their effective sensing applications. The sensing potential of Al-MOFs for metals ions are also evaluated in terms of quality assurance (e.g., limit of detection (LOD), linear range, and sensing medium). Finally, the current challenge and outlook for Al-MOFs in this research field are also outlined. This study is expected to inspire researchers in various fields to develop
Table 1
Al-MOF-based sensors for efficient and effective monitoring against a wide range of environmental pollutants.
2. Formulation of Al-MOF sensors
2.1. Direct synthesis routes of Al-MOFs
The structure and properties of Al-MOFs are highly dependent on their synthesis methods. To date, a variety of direct synthesis strategies have been reported for the fabrication of Al-MOFs. In the present section, the direct synthesis methods of Al-MOFs (e.g., solvo-/hydrothermal, reflux method, microwave-assisted, sonochemical, mechanochemical, and electrochemical approach) are discussed in relation to their merits and disadvantages (Table 1). In addition, those routes for synthesis are also summarized to specify the experimental conditions for diverse case studies (Table 2).
2.1.1. Solvo-/hydrothermal method
The solvothermal method is one of the most commonly used procedures for the preparation of Al-MOFs (Table 2) (Stock, 2014). This method employs organic solvents for preparing a suspension of the precursors. In case of the hydrothermal method, water is used as a solvent for suspending the precursors. In this method, the reactions between organic ligands and inorganic groups of Al-MOFs are usually carried out in a stainless steel autoclave at autogenous high pressure and high temperature (up to 300 ℃) for several hours (or days) (Li et al., 2020b). The properties of the Al-MOFs obtained through the solvo-/hydrothermal method significantly depend on the reaction parameters (e.g., pH value, solvent, and reaction temperature). For instance,
A summary of Al-MOF synthesis protocols along with their advantages and disadvantages.
No. Synthesis methods Energy sources Reaction time Advantages Disadvantages
1 Solvo/hydrothermal method Electrical heating Hours to days - Common synthesis of most MOFs, - Frequently used for single MOF crystals, - Easy reaction conditions to control MOF architectures (e.g., size, topology, and crystallinity)
2 Reflux method Electrical heating Hours to days -Simple and less technical instruments, -Reaction at atmospheric pressure, -Low energy consumption, -Easily synthesis procedure
3 Microwave-assisted method Microwave irradiation Minutes to hours -Fast reaction rates, -Reduced reaction time, -Less chemical waste, -Controlled properties of MOFs(in terms of porosity and size), -Phase selectivity
4 Sonochemical method Ultrasonic wave
5 Electrochemical method Electrical current
6 Mechanochemical method Mechanical force
Minutes to hours -Fast reaction rates, -Simple and energy-saving, -Ease of operation, -Mild reaction condition, -Easy formation of nanoscale MOFs in different forms(e.g., sphere morphology)
Minutes to hours
-Short reaction time, -No side reaction or impurity anions associated with metal salts, -Possibility of continuous reactions for relatively large scale production,
Hours -Simple procedure, -Eco-friendly, -Organic solvent-free process, -Fast reaction rate
-Requirement of soluble precursors, -Long reaction time, -High energy consumption, -High solvent waste generation, -Low reaction rates, -Not suitable for heatsensitive solvents and reagents
References
(Steenhaut et al., 2021; Khan et al., 2021; Yang et al., 2016)
-Long reaction time (Khan et al., 2021; Lenzen et al., 2019; Schlusener et al., 2019)
-Difficulty regulating irradiation power, -Unable to achieve the same conditions in diverse instruments, -Concerns about reproducibility
-Need for probe ultrasonic instruments, -Difficulty in accurately controlling reaction temperature
-Undesirable accumulation of MOF crystals, -High cost production, -High energy consumption
-Low crystallinity, -Occurrence of side reaction
(Vinu et al., 2017; Isaeva et al., 2015; Khan et al., 2012; Halis et al., 2015)
(Al-Attri et al., 2022; Sud and Kaur, 2021; Kim et al., 2020)
(Kalhor et al., 2021; Martinez Joaristi et al., 2012; Ghoorchian et al., 2020)
(Rubio-Martinez et al., 2017; Crawford et al., 2015; Li et al., 2021)
Reference [A] Solvo/ hydrothermal method
1 CAU-1 AlCl3 6 H2O Aminoterephthalic acid 125
2 CAU-1-NH2 AlCl3⋅6 H2O Aminoterephthalic acid 115–
3 CAU-1-(OH)2 AlCl3⋅6 H2O 2,5-dihydroxyterephthalic < 130 4
4 CAU-3 Al(NO3)3 9 H2O Terterephthalic acid 125 12
5 CAU-3-NH2 Al(NO3)3 9 H2O 2-aminoterephthalic acid 125 12
6 CAU-3-NDC AlCl3 6 H2O 2,6-naphtalenedicarboxylic acid 130 1
7 CAU-4 Al(NO3)3⋅9 H2O 1,3,5-benzenetrisbenzoate 180 24
(Ahnfeldt et al., 2009)
(Ahnfeldt and Stock, 2012)
– – (Ahnfeldt and Stock, 2012)
NaOH – BET: 1550 m2g 1 (Reinsch et al., 2012a)
NaOH – BET: 1250 m2g 1 (Reinsch et al., 2012a)
NaOH – BET: 2320 m2g 1 (Reinsch et al., 2012a)
47 BET: 1520 m2g 1 (Reinsch et al., 2012a)
8 CAU-6 AlCl3 6 H2O 2-aminoterephthalic acid 120 12 2-propanol BET: 620 m2g 1 (Reinsch et al., 2012b)
9 CAU-8 Al2(SO4)3 18 H2O 4,4′ -benzophenonedicarboxylic acid
10 CAU-9 Al(NO3)3 9 H2O 1,2,4,5-tetrakis-(4carboxylatophenyl)-benzene
m2g 1 (Reinsch et al., 2013a)
(Krüger et al., 2015)
et al., 2015)
Al(OH)3/Al2O3/ boehmite/ Al (NO3)3 9 H2O
25
26
27
28
(Loiseau et al., 2004)
(Cheng et al., 2013)
(Kim et al., 2012)
(Stavitski et al., 2011)
(Loiseau et al., 2005) 30
31
32
(Loiseau et al., 2006)
– Cetyltrimethylammonium bromide as surfactant;
1970 m2g 1 (Seoane et al., 2015)
(Barth et al., 2015) 33
1 (Yang et al., 2016)
(Stavitski et al., 2011)
35 NH2-MIL-101 AlCl3
(Serra-Crespo et al., 2011) 36
37
(Volkringer et al., 2007)
(Volkringer et al., 2013)
(Volkringer et al., 2009a) 39
(Volkringer et al., 2009a)
(Volkringer et al., 2010a)
(Volkringer et al., 2009b)
(Volkringer et al., 2010b) 43 MOF-253 AlCl3 6 H2O 2,2′ -bipyridine-5,5 -dicarboxylic acid
44 MOF-467 Al(NO3)3 9 H2O 4,4′ ,4′ ’-[benzene-1,3,5-triyl-tris (oxy)]tribenzoic acid
45 MOF-519 Al(NO3)3 9 H2O 4,4′ ,4′ ’-[benzene-1,3,5-triyl-tris (oxy)]tribenzoic acid
46 MOF-520 Al(NO3)3 9 H2O 4,4′ ,4′ ’-[benzene-1,3,5-triyl-tris (oxy)]tribenzoic acid
47 DUT-4 Al(NO3)3⋅9 H2O 2,6-naphthalene dicarboxylic acid
48
49 [Al3(OH)3(HTCS)2] Al(NO3)3 9 H2O
72
m2g 1 (Bloch et al., 2010)
76 BET: 725 m2g 1 (Wang et al., 2015a)
m2g 1 (G´ andara et al., 2014)
– – (Lee et al., 2016)
1 (Senkovska et al., 2009)
1 (Senkovska et al., 2009)
BET: 11 m2g 1 (continued on next page)
tetrakis(4-oxycarbonylphenyl) silane
50 [Al5O2(OH)3(TCS)2(H2O)2] Al(NO3)3 9 H2O tetrakis(4-oxycarbonylphenyl) silane 120
51 Al(OH)(1,4-NDC)⋅2 H2O Al(NO3)3⋅9 H2O 1,4-naphthalenedicarboxylic acid 180 24
52 [Al(OH)(hfipbb)] Al(NO3)3 9 H2O 4,4′ -hexafluoroisopropylidene-bis(benzoic acid) 160 72
53 [Al(OH)(SDC)] AlCl3 4,4′ -stilbenedicarboxylic acid 180 72
54 [Al2(OH)2(C16O8H6)] (H2O)6 Al(NO3)3 9 H2O Biphenyl-3,3′ ,5,5′ -tetracarboxylic acid 210 72
[B] Reflux method
hydrofluoric acid (Guo et al., 2017)
1 (Guo et al., 2017)
(Comotti et al., 2008)
94 – (Aguirre-Díaz et al., 2015)
1 (Lo et al., 2013)
as additive (Yang et al., 2012a)
55 CAU-10-H Al2(SO4)3/NaAlO2 Sodium isophthalate refluxing temperature 10 Ethanol 95 – (Lenzen et al., 2018
56 CAU-10-H Al2(SO4)3 18 H2O 1,3-benzene dicarboxylic acid refluxing temperature 117
91 BET: 564 m2g 1 (Frohlich et al., 2016)
57 CAU-23 NaAlO2⋅0.2H2O 2,5-thiophenedicarboxylic acid refluxing temperature 6 Water 54 BET: 1056 m2g 1 (Schlüsener et al., 2020)
58 CAU-23 AlCl3/NaAlO2 2,5-thiophenedicarboxylic acid refluxing temperature 6 Water/NaOH 84 – (Lenzen et al., 2019)
59 MIL-68 AlCl3 6 H2O 1,4-benzene dicarboxylic acid 125 18 DMF – BET: 976 m2g 1 (Tehrani and Zare-Dorabei, 2016b)
60
61
62 NH2-MIL-68
63 MIL-160 AlCl3 6 H2O 2,5-furandicarboxylic
64
65 MIL-160 AlCl3⋅6 H2O
66 MIL-160 AlCl3 2,5-furandicarboxylic acid
67 DUT-5 AlCl3 20-sulfone-biphenyl-4,40-dicarboxylic acid
68 [Al(OH)(C6H3NO4)] nH2O Al2(SO4)3 18 H2O 2,5-pyrpyrroledicarboxylic acid
[C] Microwaveassisted method
69 CAU-13 AlCl3 6 H2O Trans-1,4-cyclohexanedicarboxylic acid
70 MIL-53 Al (NO3)3 9 H2O 1,4-benzenedicarboxylic acid
71 MIL-53 Al (NO3)3 9 H2O 1,4-benzenedicarboxylic acid 130 3
72 NH2-MIL-53 Al (NO3)3 9 H2O 2-aminoterephthalic acid
73 MIL-96 Al (NO3)3⋅9 H2O 1,3,5-benzenetricarboxylic acid
75 MIL-100 Al (NO3)3 9 H2O
77 MIL-100 Al (NO3)3⋅9 H2O
80 MIL-110 Al (NO3)3⋅9 H2O
–
– – (Yang et al., 2012b)
976 m2g 1 (Tehrani and Zare-Dorabei, 2016a)
m2g 1 (Rahmani et al., 2022)
(Cadiau et al.,
(Tannert et al., 2019)
– (Henry and Samokhvalov, 2022)
1150. m2g 1 (Permyakova et al., 2017)
1260 m2g 1 (Raja et al., 2015)
93 BET: 1130 m2g 1 (Cho et al., 2020)
(Niekiel et al., 2014)
69 MW power/frequency: 1200 W/ 2.45 GHz, BET: 1403 m2g 1 (Vinu et al., 2017)
68–86
power: 200–400 W, BET: 716–990 m2g 1 (Sun et al., 2022)
et al., 2017)
et al., 2017)
m2g 1 ,
0.7 (García M´ arquez et al., 2012)
MW power: 1200 W, BET: 639 m2g 1 , (Khan et al., 2012) (continued on next page)
Table 2 (continued )
81 DUT-4 Al (NO3)3 9 H2O 2,6-napthalenedicarboxylic acid 120 0.77
82 DUT-4-(NO2)2 AlCl3⋅6 H2O 1,5-dinitro-3,7-naphthalenedicarboxylic acid 140 4
83 DUT-5 Al (NO3)3 9 H2O 4,4 -biphenyldicarboxylic acid 120 0.77
84 DUT-5-NO2 AlCl3 6 H2O 3-nitro-4,4 -biphenyldicarboxylic acid 150 3
85 DUT-5-NH2 AlCl3⋅6 H2O 3-amino-4,40-biphenyldicarboxylic acid
86 DUT-5-SO2 Al (ClO4)3 9 H2O 5,5-dioxo-dibenzo[b,d]thiophen-3,7dicarboxylic acid
1
87 [Al2(OH)2(C16O8H6)] (H2O)6 AlCl3⋅6 H2O Biphenyl-3,3′ ,5,5 -tetracarboxylic acid 210 0.17
[D] Sonochemical method
91 MW power/frequency: 1200 W/ 2.45 GHz, BET: 1184 m2g 1 (Vinu et al., 2017)
– BET: 578 m2g 1 (Halis et al., 2015)
MW power/frequency: 1200 W/ 2.45 GHz, BET: 1157 m2g 1 (Vinu et al., 2017)
– BET: 1677 m2g 1 (Halis et al., 2015)
– BET: 1966 m2g 1 (Halis et al., 2015)
– BET: 1530 m2g 1 (Halis et al., 2015)
83.5 MW power: 300 W, BET: 1272 m2g 1 (Thomas-Hillman et al., 2019)
88 MIL-53 Al(NO3)3⋅9 H2O 1,4-benzenedicarboxylic acid 150 6 Water – Sonication power: 24 kHz for 1 h, BET: 872 m2g 1 (Al-Attri et al., 2022)
89 MIL-53 Al(NO3)3 9 H2O 1,4-benzenedicarboxylic acid 220 24 Water – Sonication power: 24 kHz for 0.5 h, BET: 1538.6 m2g 1 (Ahadi et al., 2022)
90 Al fumarate Al2(SO4)3 18 H2O Fumaric acid – 0.02–0.5 Water/NaOH – Sonication power: 500 W and 20 kHz, BET: 1100–1169 m2g 1 (Kim et al., 2020)
[E] Electrochemical method
91 MIL-53 Aluminum plates Terephthalic acid 20–90 – Water/DMF – KCl as electrolyte, current density: 100 mA cm 2 , BET: 1243 m2g 1 (Martinez Joaristi et al., 2012)
92 NH2-MIL53 Aluminum plates 2-aminoterephthalic acid room temperature 0.5 Water/ ethanol – KNO3 as electrolyte, current density: 10 mA cm 2 , BET: 205 m2g 1 (Kalhor et al., 2021)
93 NH -MIL53 Aluminum plates 2-aminoterephthalic acid 90 – Water/DMF – KCl as electrolyte, current density: 10 mA cm 2 , BET: 788 m2g 1 (Martinez Joaristi et al., 2012)
94 MIL-100 Aluminum plates 1,3,5-benzenetricarboxylic acid > 60 1 Water/ ethanol – KCl as electrolyte, current density: 10 mA cm 2 (Martinez Joaristi et al., 2012)
[F] Mechanochemical method
95 Al(fumarate)OH) Al2(SO4)3 18 H2O Fumaric acid 150 – – – NaOH as co-reactant, screw speed: 55 rpm, feed rate: 10 g min 1 , BET: 1010 m2g 1 (Crawford et al., 2015)
controlling the pH in a hydrothermal reaction significantly alters the topologies of the resulting Al-MOFs (Volkringer et al., 2010a). In such reactions, aluminum sources (e.g., Al(NO3)3⋅9 H2O), organic ligands (e. g., pyromellitic acid (C6H2(CO2H)4), and solvent (i.e., deionized water) are combined to react in an autoclave under various pH conditions to obtain different topologies of Al-MOFs (Volkringer et al., 2010a). Under acidic (low) pH, the formation of MIL-118 and MIL-121 occurred favorably due to the connectivity of aluminum cations in the inorganic subnetwork via μ2-hydroxo corners of AlO6 octahedra (Volkringer et al., 2010a). At higher pH, the aluminum-centered octahedra were linked to each other through common edges of two μ2-hydroxo groups to form MIL-120. In addition, the number of coordinating carboxylic oxygen atoms per pyromellitate ligands increased from 4 to 8 by adjusting the pH value (from 1.4 to 12.2), which provided more sites to connect aluminum ions (Volkringer et al., 2010a) (Fig. 3).
The selection of proper solvent and reaction temperature is also vital for the production of desired Al-MOF products. Such a relationship can be observed from the production of NH2-MIL-53 Al-MOFs using the solvothermal method (i.e., aluminum chloride as a metal salt and aminoterephthalic acid as an organic ligand) (Stavitski et al., 2011) (Fig. 4). The presence of organic solvent (e.g., dimethylformamide) promoted quick dissolution of organic linker (i.e., amino-terephthalic acid) to yield a metastable product (i.e., NH2-MOF-235(Al)) at comparatively low temperatures (Stavitski et al., 2011). In contrast, the slow dissolution rate of organic linker (amino-terephthalic acid) resulted in the formation of highly stable products NH2-MIL-53 at intermediate temperatures when the synthesis was performed using water as solvent.
Temperature also exerts a noticeable effect on the morphology of AlMOFs (e.g., MIL-53(Al)) (Taheri et al., 2018; Al-Attri et al., 2022). For instance, Al(NO3)3⋅9 H2O and 1,4-benzenedicarboxylic acid were mixed with a molar ratio of 5:2.5 in 25 ml of water. The hydrothermal reaction was conducted for 72 h at various temperatures (e.g., 150 and 220 ℃). Particle sizes of MIL-53(Al) were observed in the range of 0.77–2.19 µm at 150 ℃ In contrast, they were reduced to 0.68–1.36 µm at 220 ℃ to reflect the effects of increasing of solubility, nucleation, and crystal growth at higher temperature (Al-Attri et al., 2022). Despite various advantageous aspects of the solvo(hydro)thermal method (e.g., production of good quality MOF crystals and prevention of the volatilized toxic substance during reaction), the major drawback of the solvo (hydro)thermal method is the energy and time (hours to days) required. Solvo(hydro)thermal techniques also suffer from unobservable reaction
process (e.g., crystals growth) for synthesis of MOF materials.
2.1.2. Reflux method
As an eco-friendly and facile approach, the reflux method has also been used for the fabrication of Al-MOFs (Khan et al., 2021; Cadiau et al., 2015; Henry and Samokhvalov, 2022; Lenzen et al., 2019; Tehrani and Zare-Dorabei, 2016a). The synthesis of MOFs based on the reflux method involves a variety of solvents (e.g., water, ethanol, and N, N-dimethylformamide (DMF)) to induce chemical reactions in a reflux condenser (at a temperature of 100–130 ℃) (Table 2) (Henry and Samokhvalov, 2022; Lenzen et al., 2018; Tannert et al., 2019). Various types of Al-MOF materials such as CAU-10-H, CAU-23, MIL-160, and MIL-68 have been synthesized using the reflux method (Schlüsener et al., 2020; Tehrani and Zare-Dorabei, 2016b; Frohlich et al., 2016). For instance, the compound [Al(C6H2O4S)(OH)] xH2O (named CAU-23) was formed by a chemical reaction between AlCl3 and NaAlO2 as the aluminum sources and sodium thiophenedicarboxylate (Na2TDC) as the organic linkers in a reflux method (Lenzen et al., 2019). The as-prepared CAU-23 exhibited nano-scale particle rods (200 nm) with a BET surface area of 1250 m2g 1 (Fig. 5a and b). The rod shape was formed by alternating units of four consecutive trans and cis corner-sharing AlO6 polyhedra. Four trans and cis corner-sharing AlO6 polyhedra corresponded to the straight and helical sections of the Al-O chain, respectively. (Fig. 5(c-e)) (Lenzen et al., 2019). Likewise, MIL-160(Al) materials (surface area of 1150 m2g 1) were prepared on a large-scale of 400 g through the reflux method (Permyakova et al., 2017). This approach nonetheless seemed to require long reaction times (hours to days). Despite some flaws, the reflux method can be regarded as a potential technique for the large-scale synthesis of MOFs with many advantageous properties (e.g., simple technical instruments, reaction at atmospheric pressure, low energy consumption, and convenient synthesis procedure) (Khan et al., 2021).
2.1.3. Microwave-assisted method
The microwave-assisted method relies on the use of electromagnetic radiation (frequency range: 300 MHz – 300 GHz) to directly heat the reaction medium (Sud and Kaur, 2021). It is considered a time and energy efficient approach for the fabrication of Al-MOFs (Stock, 2014; Stock and Biswas, 2012; Vinu et al., 2017). As compared to the external heating method (e.g., conventional electrical heating), the microwave-assisted method is an efficient option to rapidly and
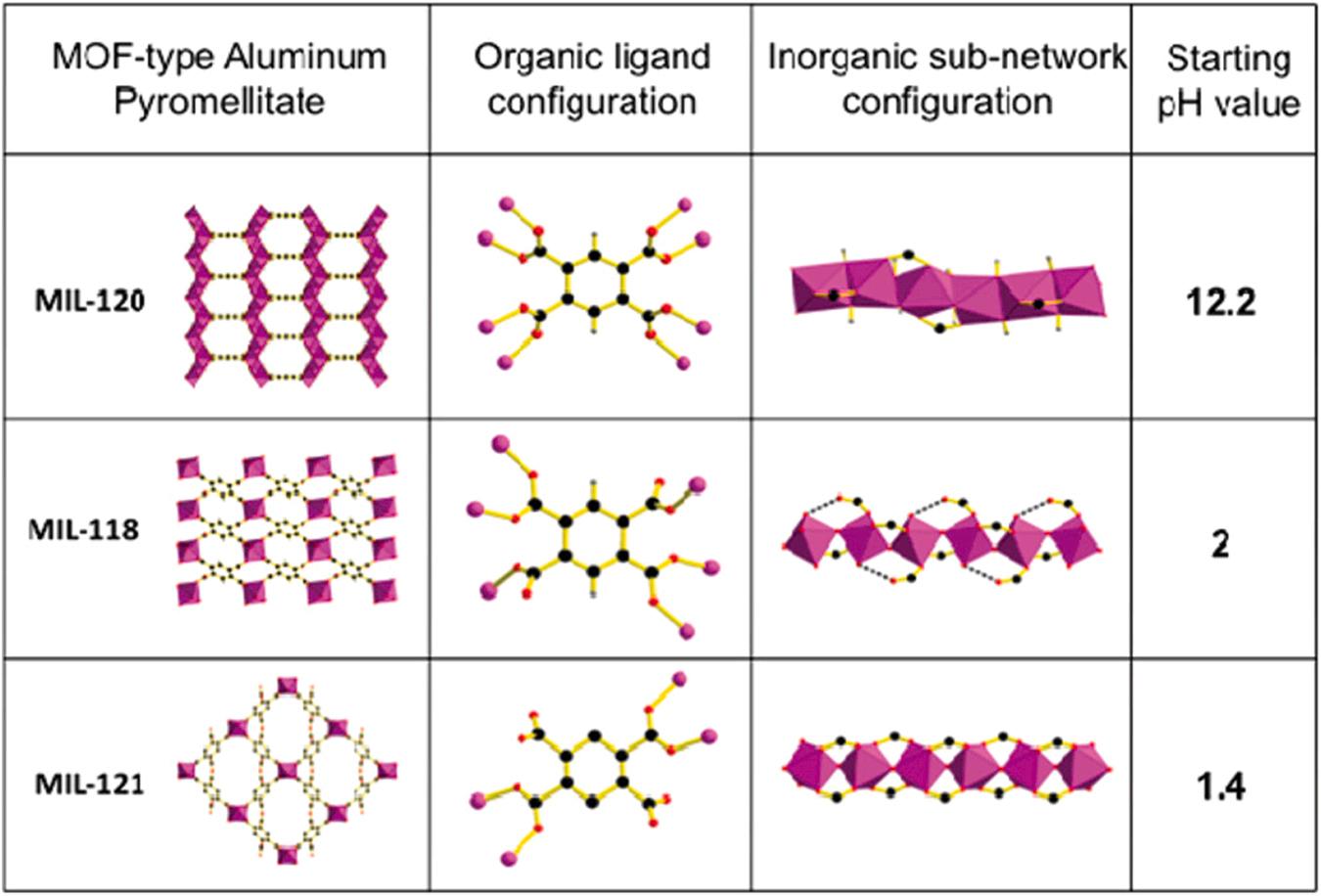
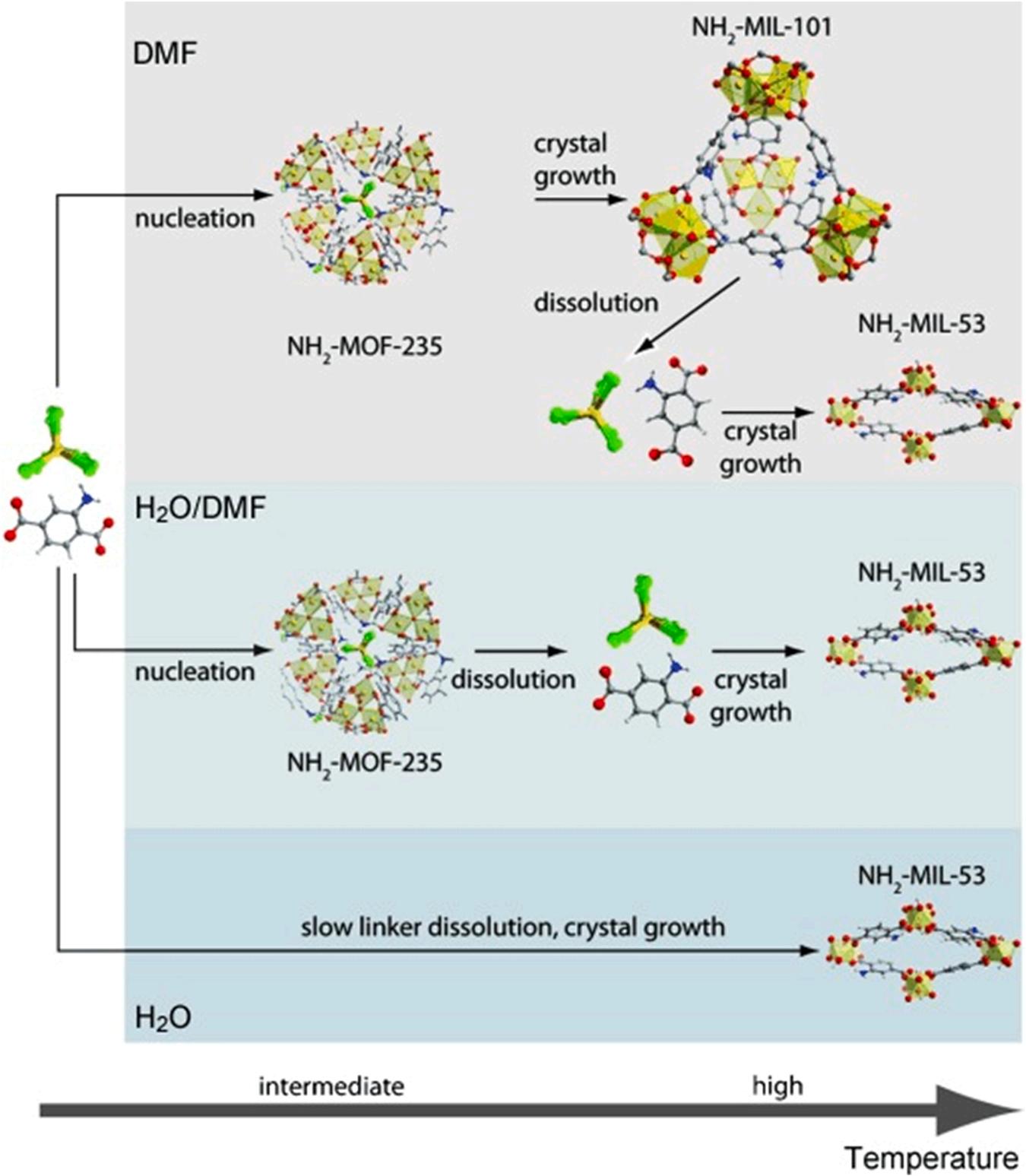
Fig. 4. Formation mechanism of NH2-MOF-235(Al), NH2-MIL-101(Al), and NH2-MIL-53(Al) in DMF/water mixtures using solvo(hydro)thermal method (Stavitski et al., 2011), copyright 2011, Wiley-VCH publishing group.
uniformly increase the temperature throughout the reaction medium (Sud and Kaur, 2021). As such, the microwave-assisted method was reported to efficiently increase the rate of the nucleation/growth process for Al-MOFs (Stock and Biswas, 2012). Therefore, the time for the induction period and complete crystallization of microwave-assisted CAU-1-(OH)2 was shortened considerably (e.g., 10–180 min) relative to conventional solvothermal method (e.g., 10–280 min) depending on the reaction temperature (Fig. 6a) (Ahnfeldt et al., 2011). In another report, the microwave method was also reported to shorten the synthesis time of NH2-MIL-101(Al) significantly from tens of hours (conventional solvothermal method) to 10–30 min (Isaeva et al., 2015). Microwave irradiation is effective for the reduction of synthesis time while serving as an effective route for determining the crystal size. As exemplified by the work of Isaeva’s group, the crystal sizes of Al-MOFs obtained through a microwave-assisted approach was smaller (e.g., 50–100 nm in case of NH2-MIL-101(Al)) compared to the solvothermal method (from 2 to 3 µm) (Isaeva et al., 2015). The small crystal size was mainly due to fast nuclei crystallization and growth during microwave reactions (Fig. 6b and c) (Isaeva et al., 2015). Accordingly, the control of key parameters (such as reaction time and microwave power) should contribute to the precise growth of crystalline Al-MOFs with well-defined geometries.
The regulation of the reaction time in the microwave-assisted method is also an effective option to obtain Al-MOF materials with desired properties (e.g., stable and high porosity or different pore sizes) (Khan et al., 2012). For instance, the porosity of
aluminum-benzenetricarboxylates (Al-BTCs) was regulated by varying the reaction time of microwave assisted synthesis approach (Khan et al., 2012). As shown in the XRD pattern and the product weight graph of MIL-96 (Fig. 6d and e), highly porous MOFs (e.g., MIL-100) were acquired at short reaction times (e.g., 1 min). In contrast, increases in the reaction time (>5 min) caused the gradual conversion of MIL-100 (less stable) into MIL-96 (more stable) (Khan et al., 2012). The short reaction time might favor the formation of kinetically controlled MOFs, while the long period promotes the production of thermodynamically stable MOFs.
Microwave power is another critical parameter to consider in the determination of the morphological features of Al-MOF materials. The effect of microwave power on the production of MIL-53(Al) was investigated (Sun et al., 2022). In this study, Al(NO3)3 9 H2O, 1,4-benzenedicarboxylate acid, and DMF/H2O were used as the aluminum source, organic ligand, and co-solvent, respectively. The mean crystal size of the obtained MIL-53(Al) was reduced from 13.26 to 10.19 nm when the applifed microwave power increased from 200 W to 400 W (Fig. 6g-h). Correspondingly, the BET surface area and pore volume decreased from 990 to 716 m2g 1 and 0.77–0.63 cm3g 1 , respectively. Such observations were accounted for by high microwave power (e.g., in terms of the aggravation of particles agglomeration, influence of nucleation, and crystal rate for MIL-53(Al) crystals) (Sun et al., 2022). Despite the advantages of microwave-assisted methods, the rising concerns of reproducibility could still be the limiting factor for its wide application (Sud and Kaur, 2021; Zhang et al., 2022a).
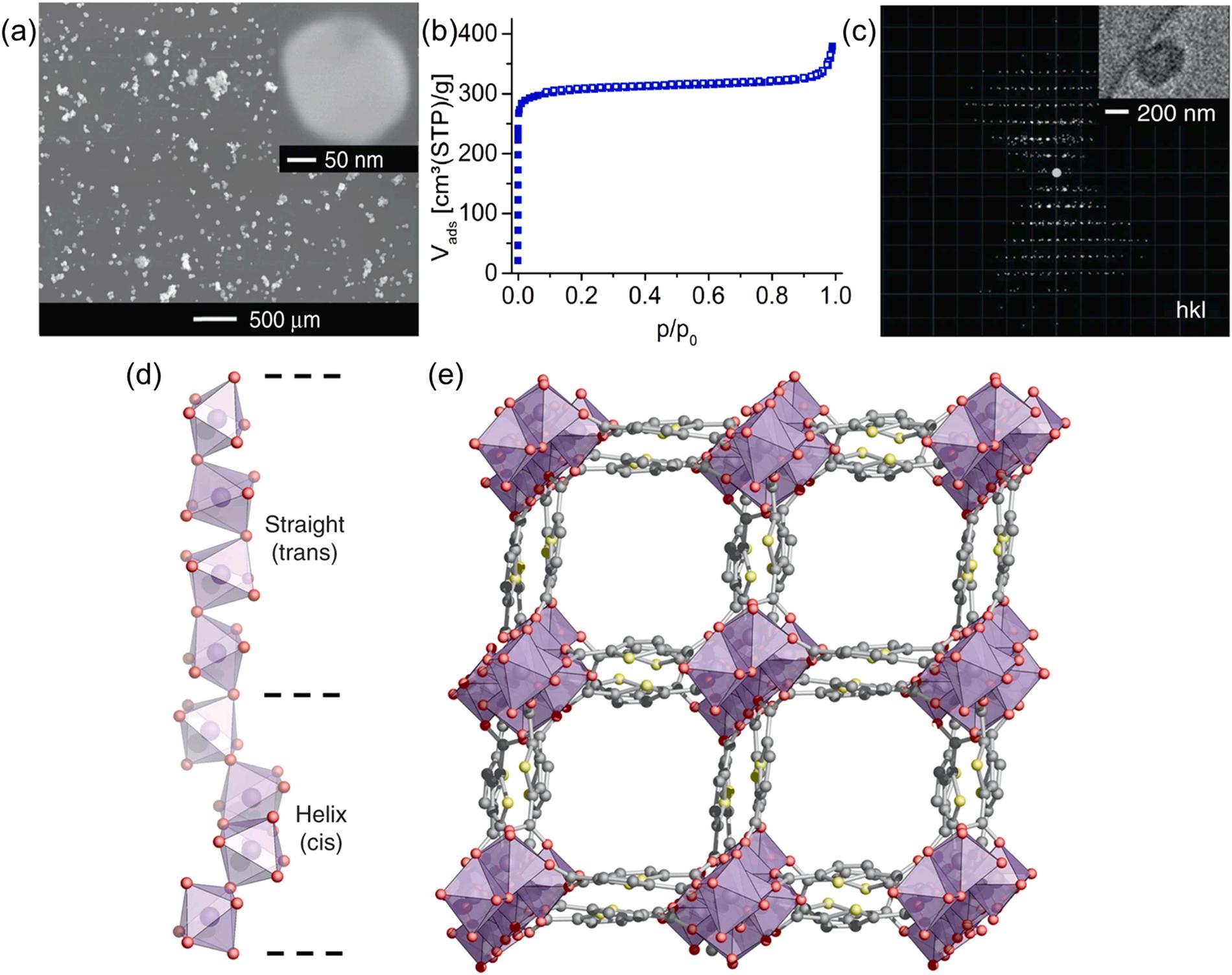
Fig. 5. The preparation of CAU-23 materials using reflux method: (a) SEM image, (b) nitrogen sorption measurement, (c) reconstructed 3D reciprocal lattice, (d) the repetition of cis and trans corner-sharing AlO6 polyhedra forming the inorganic building unit of CAU-23, and (e) the full structure of CAU-23 (Lenzen et al., 2019), copyright 2019, Springer nature publishing group.
2.1.4.
Sonochemical method
The sonochemical method has been demonstrated as an elegant synthesis route compared to the common routes (e.g., solvo/hydrothermal method) in terms of simplicity, short reaction time, ease of operation, mild reaction condition (e.g., ambient temperature), and energy-efficiency (Stock and Biswas, 2012; Safaei et al., 2019; Lee et al., 2013). The sonochemical method involves the production of bubbles in the reaction medium under high energy ultrasonic radiation (20 kHz 10 kHz) (Sud and Kaur, 2021; Stock and Biswas, 2012). The process governing the generation, growth, and collapse of bubbles is referred to as cavitation, which leads to the rapid release of energy with a high heating/cooling rates (1010 Ks 1), extreme temperature (5000 K), and high pressure (1000 atm) inside the cavitation zone as well as in its vicinity (around 200 nm) (Li et al., 2020b; Stock and Biswas, 2012). This whole process is useful for promoting chemical reactions and immediate formation of nuclei for crystallization at ambient temperature (Fig. 7a) (Kim et al., 2020). For this purpose, a solution containing an aluminum source and organic ligands was treated using an ultrasonic probe to yield highly crystallized Al-based MOFs (Fig. 7b). As displayed in Fig. 7c-d, the Al fumarate MOF prepared by the sonochemical method exhibited good sphere-like morphology. In contrast, an Al fumarate MOF synthesized by a conventional hydrothermal method showed continuously inter-grown agglomerates of spherical particles. Such good sphere-like morphology of Al fumarate MOF was attributed to the prevention of particle agglomeration by ultrasonication. As such, the BET surface area (1169 m2 g 1) of Al fumarate MOF prepared by the sonochemical
method was also found to be higher than those of the conventional hydrothermal approach (BET surface: 1078 m2 g 1) (Kim et al., 2020). Such a sonochemical method was also employed for the fabrication of other Al-MOFs (e.g., MIL-53) (Ahadi et al., 2022). Using ultrasonication, the synthesized MIL-53 also showed a higher surface area (1538.6 m2 g 1) than that of the hydrothermal method (1140 m2 g 1) (Ahadi et al., 2022; Loiseau et al., 2004). Despite the advantageous aspects of the sonochemical method (e.g., shorten reaction time and mild reaction condition), only a few studies are exclusively concerned with the sonochemical synthesis of Al-MOFs (Kim et al., 2020). More efforts should be made to expand the utilization of the sonochemical method for the preparation of Al-MOF materials.
2.1.5. Electrochemical method
The electrochemical method for the preparation of MOFs is based on applying an electric current between two electrodes immersed in an electrolyte (Fig. 8a) (Lee et al., 2013). The metal ions are continuously supplied through the metal anodic dissolution of the electrode by electrochemical oxidation. Subsequently, MOFs are formed by the reactions between these metal ions and the dissolved molecules of organic linker present in the electrolyte medium. Besides, protic solvents are used to avoid metal deposition on the electrodes (i.e., cathode). As this method can technically avoid the inclusion of anions (e.g., nitrate and chloride) from metal salts, it is advantageous for the large-scale production of pure crystalline Al-MOFs. Furthermore, the method also benefits from several advantages such as short reaction time (e.g., within minutes or hours),
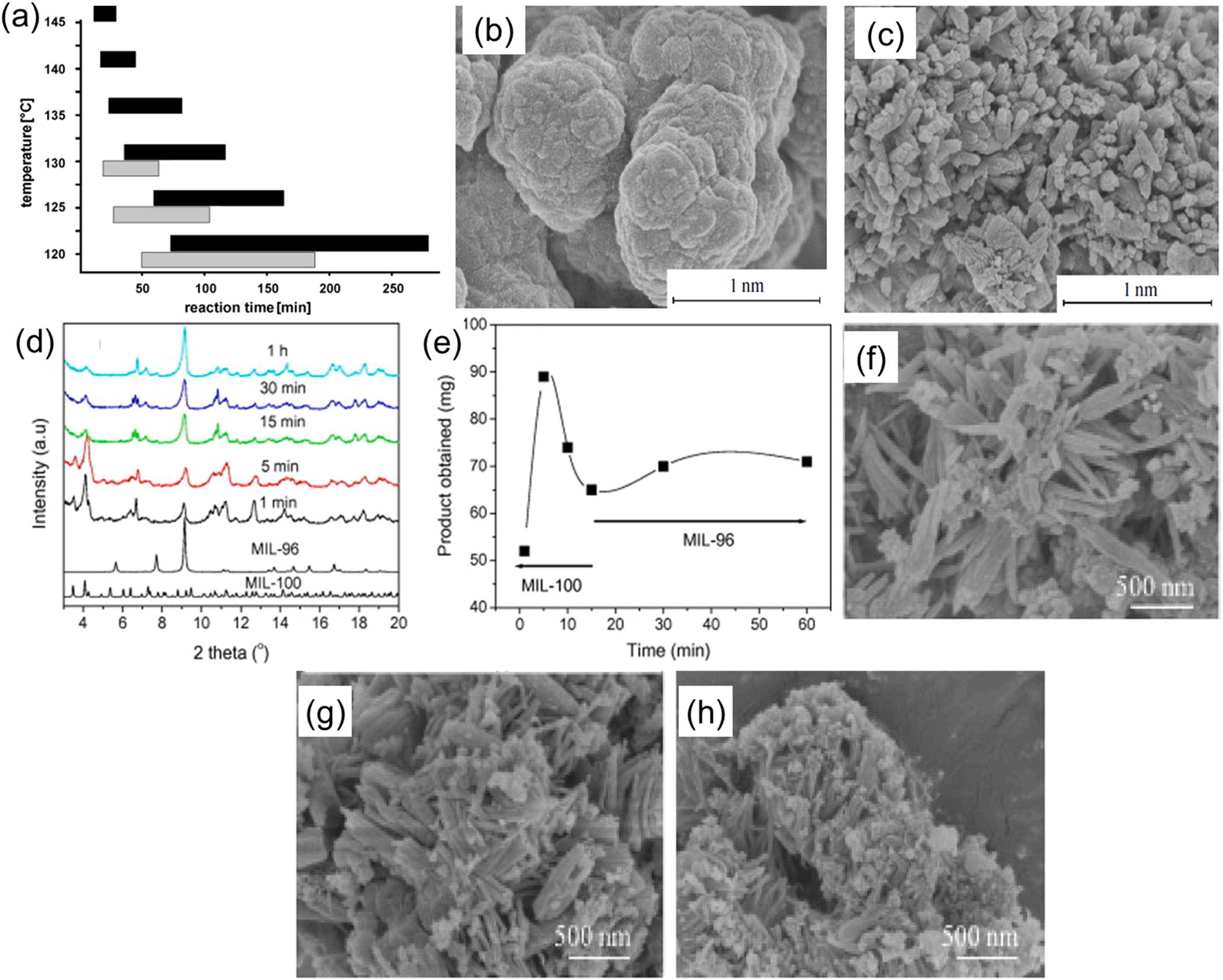
Fig. 6. The preparation of Al-MOF materials using microwave-assisted method: (a) comparison of induction and reaction time between microwave (grey) and solvothermal (black) method for production of CAU-1-(OH)2 (Ahnfeldt et al., 2011), copyright 2011, Wiley-VCH publishing group. SEM images of the NH2-MIL-101 (Al) synthesized by various methods: (b) solvothermal method and (c) microwave-assisted method (Isaeva et al., 2015), copyright 2015, Springer nature publishing group. The influence of reaction time on the production of Al-BTCs: (d) XRD patterns, and (e) obtained weight of Al-BTCs (Khan et al., 2012), copyright 2012, Elsevier publishing group. The effect of microwave power on the synthesis of MIL-53(Al): (f) 200 W, (g) 300 W, and (h) 400 W (Sun et al., 2022), copyright 2022, Elsevier publishing group.
mild reaction conditions (at ambient temperature and pressure), and scalability (Raptopoulou, 2021; Rubio-Martinez et al., 2017; Zhang et al., 2019b).
In a typical electrochemical synthesis procedure, aluminum metallic plates were inserted in the presence of organic linkers (e.g., 2-amino terephthalic acid) and an electrolyte support (e.g., potassium nitrate) in an ethanol/water (10/90, v/v) mixture under stirring (Kalhor et al., 2021). MIL-53(Al)-NH2 was successfully synthesized (Fig. 8b) on the basis of the electrochemical method. Upon establishing a current density of 10 mA cm 2 , the hydroxide ions were in-situ electro-generated on the surface of the cathode to increase the pH at the cathode surface (Fig. 8b). As such, water molecules were electro-reduced to deprotonate ligands. In addition, the anode surface was oxidized to generate aluminum cations required for the formation of MIL-53(Al)-NH2 crystals. In the electrochemical approach, pore-blocking of prepared Al-MOF was prevented due to the absence of cationic salts (required to construct MOF) (Kalhor et al., 2021). The electrochemically formulated Al-MOFs (e.g., MIL-53(Al)-NH2) generally exhibit high surface area (e.g., 204.75 m2 g 1) with a wide pore size (of 4.2 nm) (Kalhor et al., 2021). The surface
area of MIL-53(Al)-NH2 was further improved to 788 m2 g 1 using a high reaction temperature (90 ℃), high solubility solvent for linkers (DMF), and deprotonation reagent of linkers (tributylmethylammonium methyl sulfate) (Martinez Joaristi et al., 2012). In addition, this electrochemical technique can help formulate Al-MOFs with uniform structures (e.g., 3D cauliflower morphology) (Fig. 8c) (Kalhor et al., 2021). Of late, limited literature has reported on the electrochemical synthesis of aluminum-based MOFs (Kalhor et al., 2021; Martinez Joaristi et al., 2012).
2.1.6. Mechanochemical method
The mechanochemical method is an eco-friendly strategy for the large-scale production of Al-MOF materials. This approach is mainly applied to utilize MOF precursors with poor solubility (e.g., oxides, hydroxides, and carbonates) (Rubio-Martinez et al., 2017; Chen et al., 2019b). The mechanochemical method relies on both physical and chemical interactions using external mechanical force/energy (e.g., milling, grinding, and extrusion) (Stock and Biswas, 2012; Rubio-Martinez et al., 2017; Crawford and Casaban, 2016). Under such
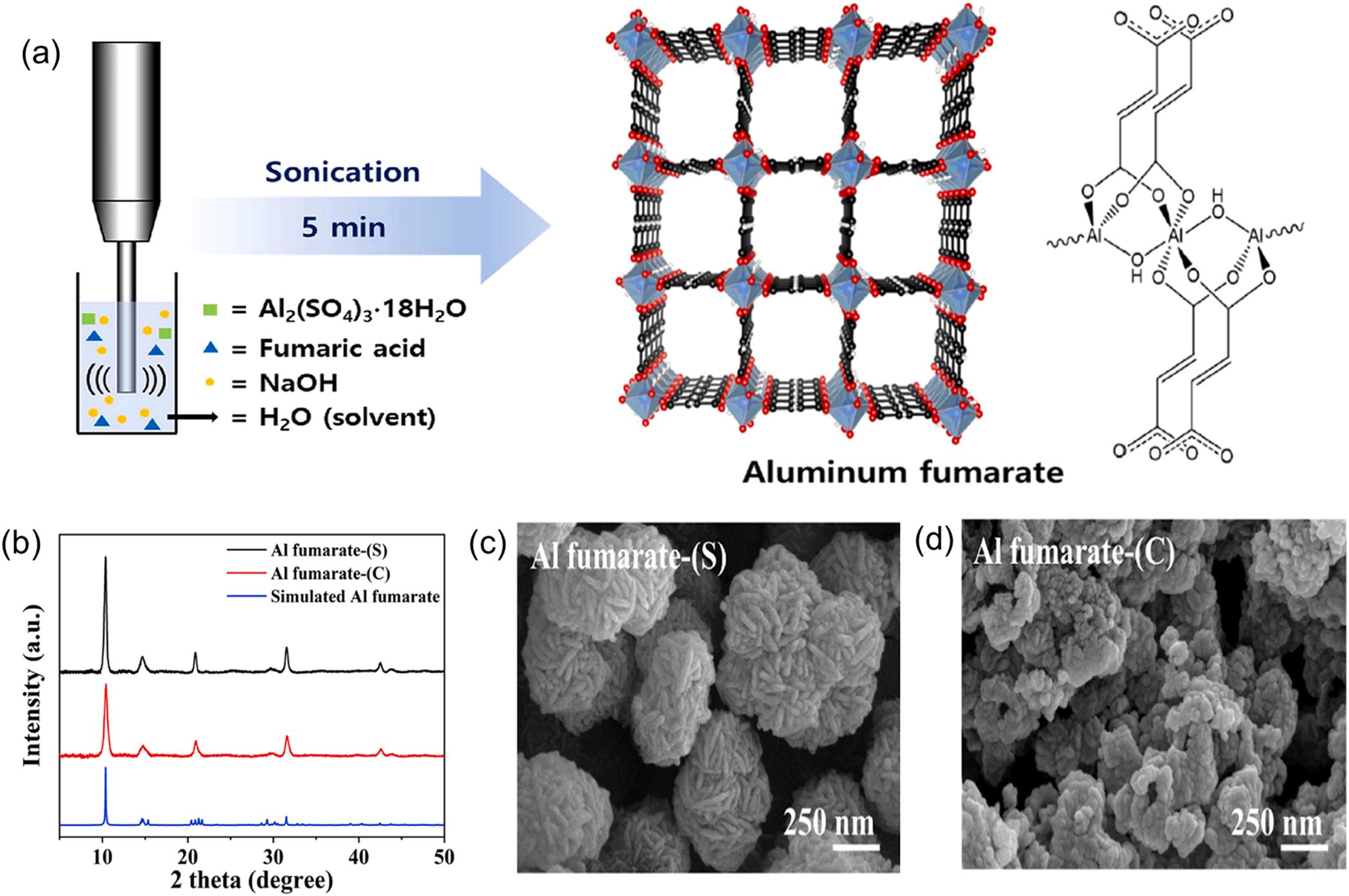
Fig. 7. The preparation of Al-MOFs materials using sonochemical method: (a) schematic illustration of preparing aluminum fumarate MOF materials, (b) PXRD patterns of aluminum fumarate MOF materials prepared by various methods: sonochemical method (black) and conventional hydrothermal method (red). SEM images of aluminum fumarate MOF materials prepared by various methods: (c) sonochemical method and (d) conventional hydrothermal method (Kim et al., 2020), copyright 2020, Elsevier publishing group.
high mechanical energy and dry conditions (i.e., without solvents), the intramolecular bonds in metal salts are mechanically disrupted to reconstruct new chemical bonds between metal cations and organic ligands (Younis et al., 2021). For example, the mechanochemical method has been used to synthesize Al(fumarate)(OH) MOF materials by external extrusion (Fig. 9a) (Crawford et al., 2015). In such cases, reactants (i.e., cation source, organic ligands, and anion (OH-) source) were mixed in the absence of solvent (Fig. 9b) (Crawford et al., 2015). The product obtained through mechanochemical method possessed BET surface areas (1010 m2 g 1) comparable to those of other methods (e.g., solvothermal method, 1020 m2 g 1) (Gaab et al., 2012; Crawford et al., 2015; Jeremias et al., 2014). Due to the lack of solvents, rapid reactions, and small free volume present in the twin screw extruder, the mechanochemical method showed an excellent process efficiency in the synthesis of Al(fumarate)(OH) MOF materials (e.g., high space time yield (STY: 27000 kg m 3 day 1) relative to a solvothermal-based process (STY: 3600 kg m 3 day 1)) (Crawford et al., 2015). Unlike traditional methods (e.g., solvothermal approach), the main benefits of the mechanochemical synthesis include its environmentally friendly nature, high production yield of Al-MOF in a short reaction period, lack of organic solvent, and cost-effectiveness (Rubio-Martinez et al., 2017). However, it should be noted that this method may also suffer from the generation of some by-products due to the interference of anionic salts. Hence, further purification is frequently required to improve the quality of the final products (Rubio-Martinez et al., 2017).
2.2. Functionalization of Al-MOFs with sensing elements
A number of strategies (e.g., utilization of stimuli responsive organic ligands during the direct synthesis of Al-MOFs, post-synthetic modification (PSM) approaches, and formation of Al-MOFs composites) can be considered to impart or improve the sensing properties of Al-MOF materials, as discussed below.
2.2.1. Utilization of functional organic ligands in direct synthesis
The basic information on the construction and luminescent mechanisms of luminescent MOFs has been explained in elsewhere (Lustig et al., 2017; Hu et al., 2014; Yang et al., 2021). The luminescent properties of MOFs can be accounted for by the combined effects of several factors such as organic ligands-metal charge transfers, the organic ligands with aromatic moieties or extended π systems, and introduced lanthanide ions (Cui et al., 2012). Luminescent Al-MOF-based sensors have commonly been built with the aid of stimuli responsive organic ligands to effectively gain the capacity for the luminescent detection towards analytes (e.g., metal ions) (Li et al., 2019; Qin et al., 2021; Yang et al., 2013). In this respect, the luminescent properties of Al-MOFs can be mainly attributed to the emission phenomena of organic ligands and ligand-metal charge transfer (Lin et al., 2020; Yang et al., 2013; Liu and Yan, 2016). Some examples of π-containing organic ligands are 2-aminoterephthalic acid, terephthalic acid, 2,2′ ,2′ -(-triazine-2,4,6-triimino)tribenzoic acid), 5-formyl isophthalic acid, and 2,5-thiophenedicarboxilic acid (Li et al., 2019; Zhu et al., 2020; Díaz-Ramírez et al., 2020; Ma et al., 2021; Nandi et al., 2019). The luminescence properties of Al-MOFs greatly rely on the π electrons of organic ligands, which help generate
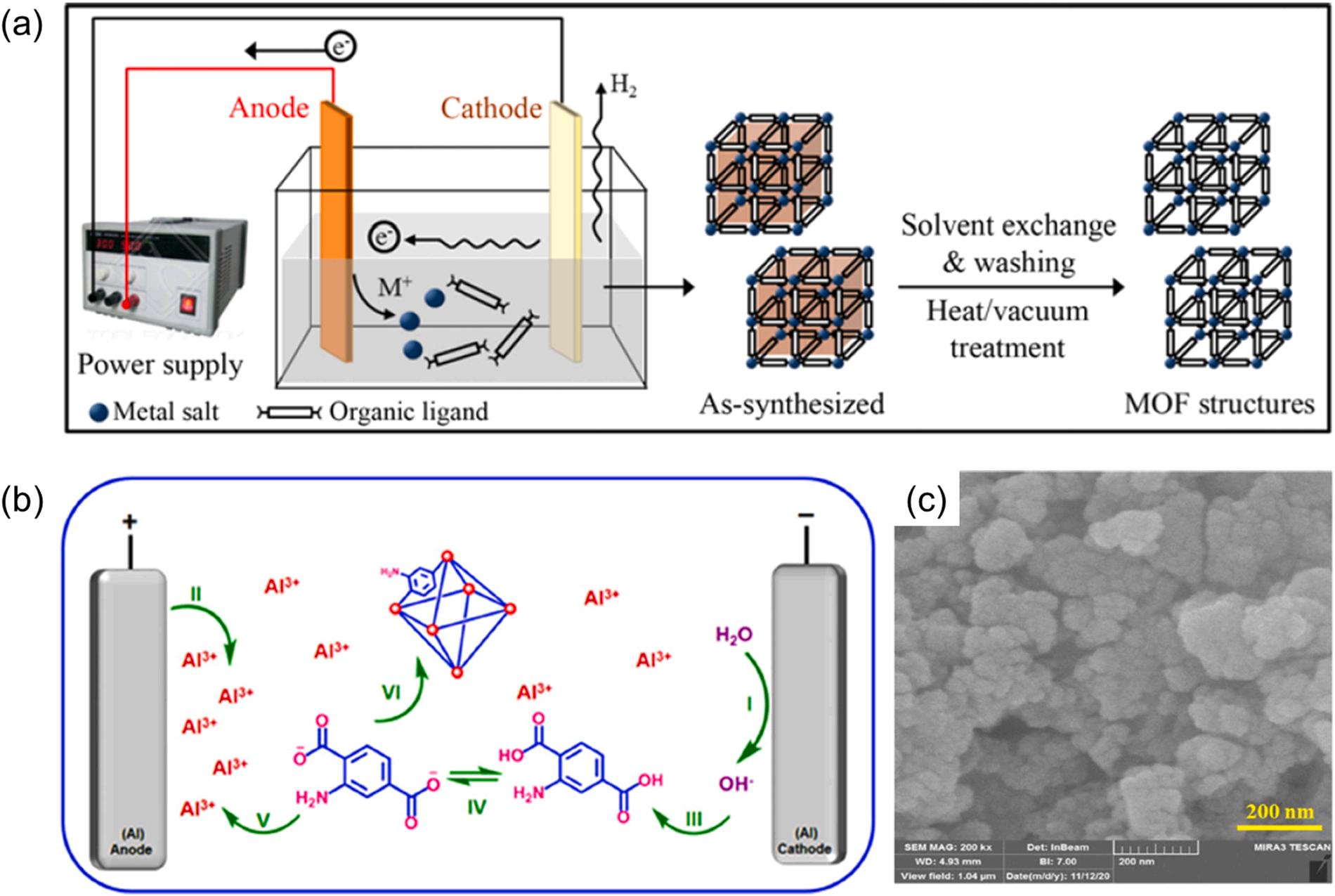
8. The preparation of Al-MOF materials using electrochemical method: (a) typical schematic of preparing MOFs (Lee et al., 2013), copyright 2013, Springer nature publishing group. (b) Fabrication of MIL-53(Al)-NH2 and (c) their SEM images (Kalhor et al., 2021), copyright 2021, Springer nature publishing group.
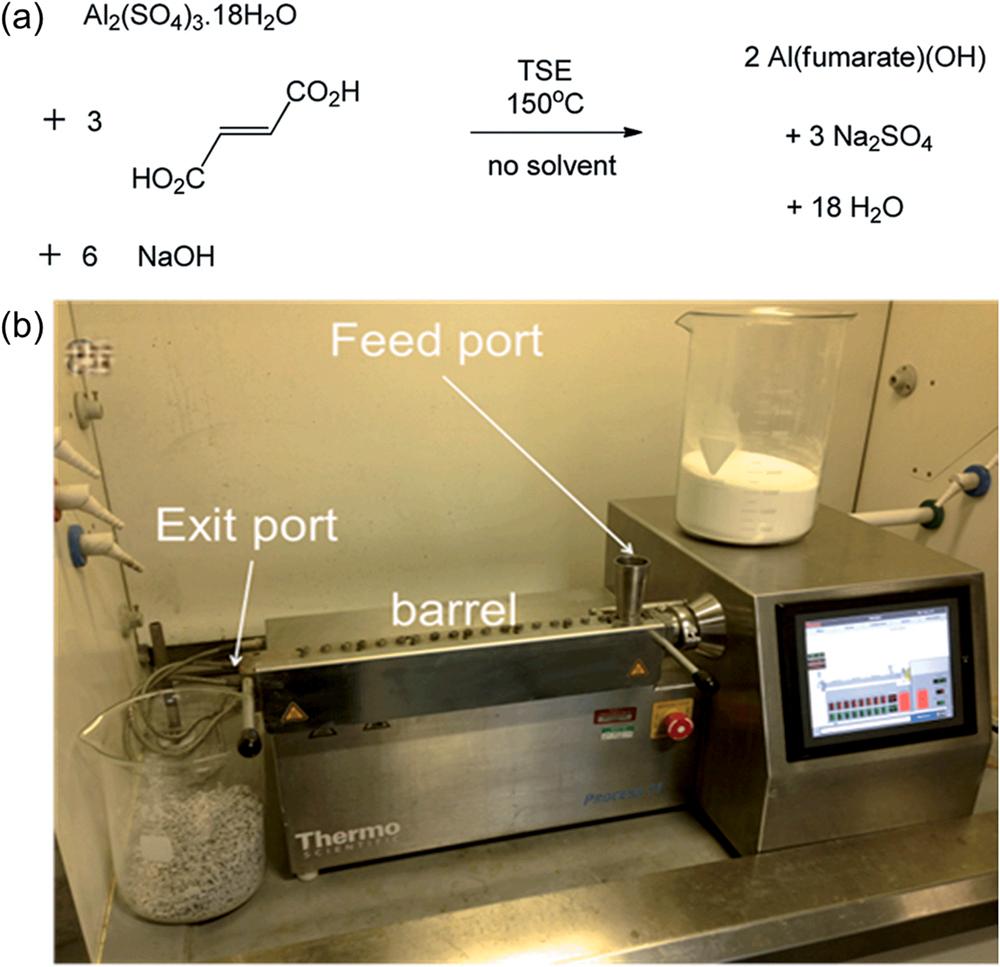
Fig. 9. The preparation of Al(fumarate)(OH) MOF materials using mechanochemical method: (a) chemical reaction and (b) the twin screw extruder instrument used (Crawford et al., 2015), copyright 2015, Royal Society of Chemistry (RSC) publishing group.
the signals for the detection process (Lin et al., 2020; Hu et al., 2014; Qin et al., 2021). For example, MIL-101(Al)-NH2 MOFs were prepared using 2-aminoterephthalic acid as an organic ligand (Lin et al., 2020). The formulated Al-MOFs exhibited luminescence properties with the
excitation of π electrons (π→π * ) of ligands (Lin et al., 2020). It is also suggested that the luminescence in Al-MOFs generates from the charge transfer between metal ions and organic ligands (Zhang et al., 2019a; Yang et al., 2013). It is recognized that the ligand-metal charge transfer should be based on the electronic transition (e.g., from an organic linker-localized orbital to a metal-centered orbital) (Cui et al., 2012). As a good example, the blue emission of NH2-MIL-53 (Al) at 450 nm under the excitation of 340 nm was attributed to the electronic transition from the 2-aminoterephthalic acid ligands to Al metal ions clusters (Xie et al., 2021). Further, ratiometric fluorescence can decrease the environmental interference and vision tiredness to provide easy-to-differentiate color and intensity changes compared with that of single-color fluorescence detection (Chen et al., 2020). As such, the construction of ratiometric fluorescent Al-MOF-based sensors can be realized through the combination of stimuli responsive and optically active ligands. In this regard, a ratiometric fluorescent ruthenium-based Al-MOF (Ru@MIL NH2) sensor was developed using stimuli responsive 2-aminoterephthalic acid organic ligands and Ru(bpy)3Cl2⋅6 H2O] as optically active organic ligands (Yin et al., 2017). Despite the facile introduction of sensing elements into a MOF architecture through a direct method, these sensors generally suffer from challenges including low linker solubility, poor thermal stability, and undesirable chemical reactions between cations and linker functional moieties (Schneemann et al., 2014; Sun and Zhou, 2015; Cohen, 2012).
2.2.2. Post-synthetic modification
The post-synthetic modification (PSM) approaches are promising options to functionalize Al-MOFs with sensing moieties. PSM helps introduce new functional groups within the main molecular skeleton of synthesized Al-MOFs while maintaining their intrinsic structure (Cohen, 2012; Mandal et al., 2021; Kamel et al., 2021; Zhang and Yan, 2019; Wang and Cohen, 2009; Yin et al., 2019; Kalaj and Cohen, 2020). Based
on the nature of bonds formed between modified reagents and MOFs, the PSM strategy can be divided into two types, i.e., dative and covalent PSMs (Cohen, 2012).
The dative PSM method is generally used to introduce luminescent compounds (e.g., lanthanide ions such as samarium (Sm3+), europium (Eu3+), dysprosium (Dy3+), erbium (Er3+), and ytterbium (Yb3+)) to the structure of Al-MOFs for sensing applications (Younis et al., 2021; Cui et al., 2014). In fact, lanthanide ions can coordinatively react with the uncoordinated active sites of the organic linkers in MOFs to form functional luminescent Al-MOFs materials (Zhang and Yan, 2019; Yan, 2017). The lanthanide ions can exhibit (characteristic) narrow 4–4 f transitions emission (Cui et al., 2012). As such, the luminescent properties of lanthanide/Al-MOFs materials should be generated from the f-f transitions of lanthanide ions (Luo et al., 2019; Dao et al., 2018). More specifically, the Eu3+@MOF-253 can exhibit several strong emission peaks locating at 579, 592, 612, and 699 nm, which correspond to the 5D0→7FJ (J=0–4) transition of Eu3+ ions (Luo et al., 2019).
On the other hand, covalent PSM of Al-MOFs is achieved through the formation of covalent bonds between functional reagents (e.g., 3-formylsalicylic acid ligand (3-FSA)) and the organic linkers of Al-MOFs (Kamel et al., 2021). For example, a luminescent Al-MOF (e.g., 3-FSA-NH2-MIL-53(Al)) was fabricated for the detection of metal ions via a Schiff base reaction. The strong covalent bond between the sensing element and the Al-MOF offered high chemical stability (robust framework) to the resulting materials (Kamel et al., 2021).
2.2.3. Formation of Al-MOF composites
Composite formulations are alternative functionalization methods to endow Al-MOFs with strong and specific sensing abilities (e.g., electrochemiluminescence and electrochemical) (Feng et al., 2021, 2020; Wang et al., 2020b; Duan et al., 2019). Quantum dots (QDs) as novel semiconductor materials are effective options to offer high quantum yield and good electrical conductivity (Aguilera-Sigalat and Bradshaw, 2016). In addition, Prussian blue (PB) consisting of Fe4[Fe(CN)6]3- exhibits unique catalytic properties (Duan et al., 2019). Carbon nanotubes (CNTs) have the unique properties of excellent conductivity (Camilli and Passacantando, 2018).
Al-MOF-based composite sensors are generally synthesized through the incorporation of nanomaterials/nanofillers (e.g., CdS quantum dots, Au nanoparticles (NPs), carbon dots (CDs), CdTe quantum dots, polypyrrole, carbon nanotubes (CNTs), and Prussian blue (PB)) on the surface or into the cavities of MOFs (Feng et al., 2021, 2020; Wang et al., 2020b; Duan et al., 2019; Xu and Yan, 2016). For example, electro-chemiluminescent Al-MOF-based composite sensors were formulated through the incorporation of CdS quantum dots (QDs) and Au nanoparticles as electro-chemiluminescence emitters (energy donor) and energy acceptors, respectively (Feng et al., 2021). In another study, carbon nanotubes and Prussian blue were combined with MIL-53(Al), and the MIL-53(Al)/CNTs/PB composite was able to generate a current response because of the electrochemical catalytic activity of PB (Duan et al., 2019).
3. Applications and sensing mechanism of Al-MOF sensors for detection of metal ions
In general, Al-MOF-based sensors are built to detect the target analytes (e.g., metal ions) based on luminescent, electrochemiluminescence, and electrochemical techniques. The strategies for using functionalized Al-MOFs for sensing of various metal ions are summarized in Table 3.
The luminescent-based Al-MOF sensors exhibited variable luminescent emission intensity, i.e., luminescence enhancement (turn-on) and quenching (turn-off) when recognizing the analytes (Shi et al., 2021b). Generally, the quenching luminescence mechanism of MOFs by metal ions includes (i) the interactions between the organic ligands and the exterior metal ions/analytes (ligand-analytes interaction) and (ii) cation
exchange between the MOFs and the external metal ions (cation exchange), or a combined mechanism (e.g., cations exchanges and ligand-analytes interactions)) (Dao et al., 2018; Liu et al., 2019; Huangfu et al., 2021; Wang et al., 2017). The quenching effect can be quantitatively obtained using the Stern-Volmer equation (Huangfu et al., 2021; Johnson et al., 1998):
I0/I=1+Ksv[M] (1)
where I0 and I are the luminescent intensities of MOFs before and after recognition of the analyte, respectively, [M] is the concentration of the analyte, and Ksv is the Stern-Volmer constant. A higher Ksv value indicates a higher efficiency of the MOF sensor (Huangfu et al., 2021). In the case of ligand-analyte interactions, the exterior metal ions/analytes coordinated with the organic ligands can change the organic properties (e.g., electron density, rigidity, or conformation), thereby resulting in luminescent quenching of MOFs (Huangfu et al., 2021; Hao et al., 2013). For example, a turn-off luminescent sensor with pyrene functionalized ligand (MIL-53-L) was designed and constructed for sensing Cu2+ in an aqueous solution (Liu and Yan, 2016). The imine part of the organic ligand (i.e., 2-(pyrene-1-imine) terephthalic acid (H2L)) offered binding sites for Cu2+ ions, while its pyrene moieties acted as fluorophores. The Cu2+ ions effectively quenched the luminescence emission at 567 nm of MIL-53-L relative to other metal ions (e.g., Hg2+ , Zn2+ , Mn2+ , and Fe2+) under excitation at 337 nm. In such cases, N atoms of the internal imine groups interacted with Cu2+ ions to convert them into amide groups. MIL-53-L showed a linear quenching range of 0–500 µM with a high Ksv value of 6.15 × 103 µM 1 towards the detection of Cu2+ ions (Liu and Yan, 2016).
The cation exchange mechanism in luminescent MOFs having a neutral framework is based on the exchange of the analyte metal ions and post-modified framework of MOFs (Yang et al., 2013; Huangfu et al., 2021; Dao and Ni, 2017). For instance, the cation exchange between Fe3+ and the framework Al3+ ions in MIL-53(Al) caused fluorescence quenching at 410 nm emission in MIL-53(Al) under an excitation wavelength at 305 nm (Fig. 10a) (Yang et al., 2013). Such a phenomenon was attributed to the transformation of strong-fluorescent MIL-53 (Al) to weak-fluorescent MIL-53(Fe) (Fig. 10b). As a result, this MIL-53 (Al) sensor allowed sensitive detection of Fe3+ ions in an aqueous solution with a linear range of 3–200 µM (LOD of 0.9 µM) (Yang et al., 2013). In another study, post-synthetically modified Al-MOFs with Tb3+ ions (i.e., MIL-110(Al)/Tb3+) were constructed to sense Fe3+ ions (Dao and Ni, 2017). The fluorescence response of MIL-110(Al)/Tb3+ toward target metal ions was validated for its potential use for metal ion detection. Fig. 10c shows that only Fe3+ ions can fully quench the fluorescence emission in MIL-110(Al)/Tb3+ , implying its high Fe3+ ion selectivity. Herein, MIL-110(Al)/Tb3+ was used for detection of Fe3+ ions with a wide linear range (0–500 µM), low detection limit (655 nM), and high quenching constant (Ksv: 6.177 ×103 µM 1) using 301 nm excitation (Fig. 10d). As confirmed by EDS and ICP technologies, the luminescent quenching of MIL-110(Al)/Tb3+ toward Fe3+ ions was accounted for by cation exchange between Fe3+ and Tb3+ ions. In addition, the MIL-110(Al)/Tb3+ exhibited high selectivity against Fe3+ ions in aqueous solution due to the stronger affinity of the –COOH group to Fe3+ ions (Dao and Ni, 2017).
In some cases, the fluorescence quenching phenomena are complex and may involve the combination of several quenching mechanisms (Lustig et al., 2017; Gao et al., 2018; Das et al., 2018). For example, aqueous phase sensing of bismuth ions (Bi3+) using [Al8(OH)4(OCH3)8(BDC(OH)2)6] x H2O (CAU-1-(OH)2) was achieved based on cation exchange and the ligand-analyte interaction mechanisms (Gao et al., 2018). CAU-1-(OH)2 emits bright green fluorescence at 505 nm upon excitation of 365 nm in water due to the intramolecular proton transfer process between the ligand and water molecule (Jayaramulu et al., 2010). Further, among various metal ions (e.g., Na+ , K+ , Ba2+ , and Ca2+), the specificity of CAU-1-(OH)2 towards Bi3+ ions was explained
Table 3
Summary of Al-MOF-based sensors employed for the detection of various metal ions.
No. Al-MOF materials Sensing approach/type
Fe3þ ions
1 MIL-53(Al) Luminescence/turn off Cation exchange Aqueous solution
2 Al-MIL-53-N3 Luminescence/turn off Cation exchange/ ligand-analyte interactions Water
3 MIL-53(Al)/Eu3þ Luminescence/turn off Cation exchange Aqueous solution
4 MIL-110(Al)/ Tb3þ Luminescence/turn off Cation exchange Aqueous solution 0–
5 Eu3þ@MIL53–COOH(Al) Luminescence/turn off Cation exchange Aqueous solution
6 Al-MOF (tricarboxylate ligands) Luminescence/turn on
[B] Cu2þ ions
7 MIL-53-L Luminescence/turn off Ligand-analyte interactions Aqueous solution
8 Eu3þ@MOF-253 Luminescence/turn off Ligand-analyte interactions Aqueous
(Dao et al., 2018)
and Ni, 2017)
+ (Zhou et al., 2014)
, Cu+ ,
+ , Mg2+ , Ba2+ ,
+ , Ni2+ , Sn2+ , Fe3+ , and Sr2+ (Ma et al., 2021)
+ ,
+ ,
+ , and
+ ,
+ (Liu and Yan, 2016)
+ , K+ ,
+ , Cr3+ ,
+ ,
+ , Ni2+ , Zn2+ , Cd2+ , and Pb2+ (Luo et al., 2019)
9 NH2-MIL-101(Al) @ZIF-8 Luminescence/turn off Ligand-analytes interactions Aqueous solution 1.5–625 0.17 Li+ , Na+ , K+ , Ca2+ , Mg2+ , Mn2+ , Co2+ , Ni2+ , Hg2+ , Pb2+ , and Cd2+ (Zhang et al., 2018b)
10 NH2-MIL-53(Al)/ Ppy Electrochemical Differential pulse voltammetry Aqueous solution 0.015–6.294 3.84 × 10 3 Cd2+ , Hg2+ , Ca2+ , Ni2+ , and Zn2+ (Wang et al., 2020b)
[C] Hg2þ ions
11 NH2-MIL-53(Al) Luminescence/turn off – Aqueous solution 0.002–38.27 0.23 × 10 3 Zn2+,Ca2+ , Mn2+ , Cd2+ , Mg2+ ,
+ ,
+ ,
+ , Pb2+ , Cr3+ ,
+ ,
+ , rutin, Al3+ , sucrose, glucose, and palmatine (Ren et al., 2020)
+ ,
12 NH2-MIL-53(Al) Luminescence/turn off Ligand-analyte interactions Aqueous solution
13 MIL-53(Al)
14 Eu3þ/ CDs@MOF-253 Ratiometric and colorimetric
[D] Pb2þ ions
15 MIL-53(Al) @CdTe-PEI
16 NH2-MIL-53(Al)/ Ppy Electrochemical Differential
[E] Pd2þ ions
+ , K+ ,
+ ,
+ , Co2+ , Ca2+ , Pb2+ , Cd2+,Zn2+ ,
+ , and
+ (Zhang et al., 2019a)
(Feng et al., 2020)
(Xu and Yan, 2016)
+ , and
+ (Wang et al., 2020b)
19 CAU-10-V-H Luminescence/turn off Ligand-analyte interactions Water – 0.11 Ag+ , Cr3+ , Cu2+ , Cd2+ , Co2+ , Eu3+ ,
20 Al-CAU-10OC3H5 Luminescence/turn off Ligand-analyte interactions Water –
[F] Agþ ions
17 Sm3þ@MIL-121 Luminescence/turn on Antenna effect Aqueous solution 0–500
Na+ ,
+ ,
+ ,
+ , and Zn2+ (Nandi et al., 2020)
+ (Ghosh et al., 2021)
+,Ca2+ ,
+ , Mn2+ ,
+ ,
+ , Cu2+ , Ag+ , Zn2+ , Cd2+ , and Pb2+ (Hao and Yan, 2015) (continued on next page)
+ ,
Table 3 (continued )
18 Eu3þ@MIL-121 Luminescence/turn on Antenna
[G] Bi3þ ions
21 CAU-1-(OH)2
[H] Fe2þ ions
Luminescence/turn off Cations exchange/ ligand-analyte interactions Aqueous solution
22 MOF-253 Luminescence/turn off Ligand-analyte interactions Aqueous solution
*The selectivity is the ability of sensors to recognize the target analytes in the presence of interferences.
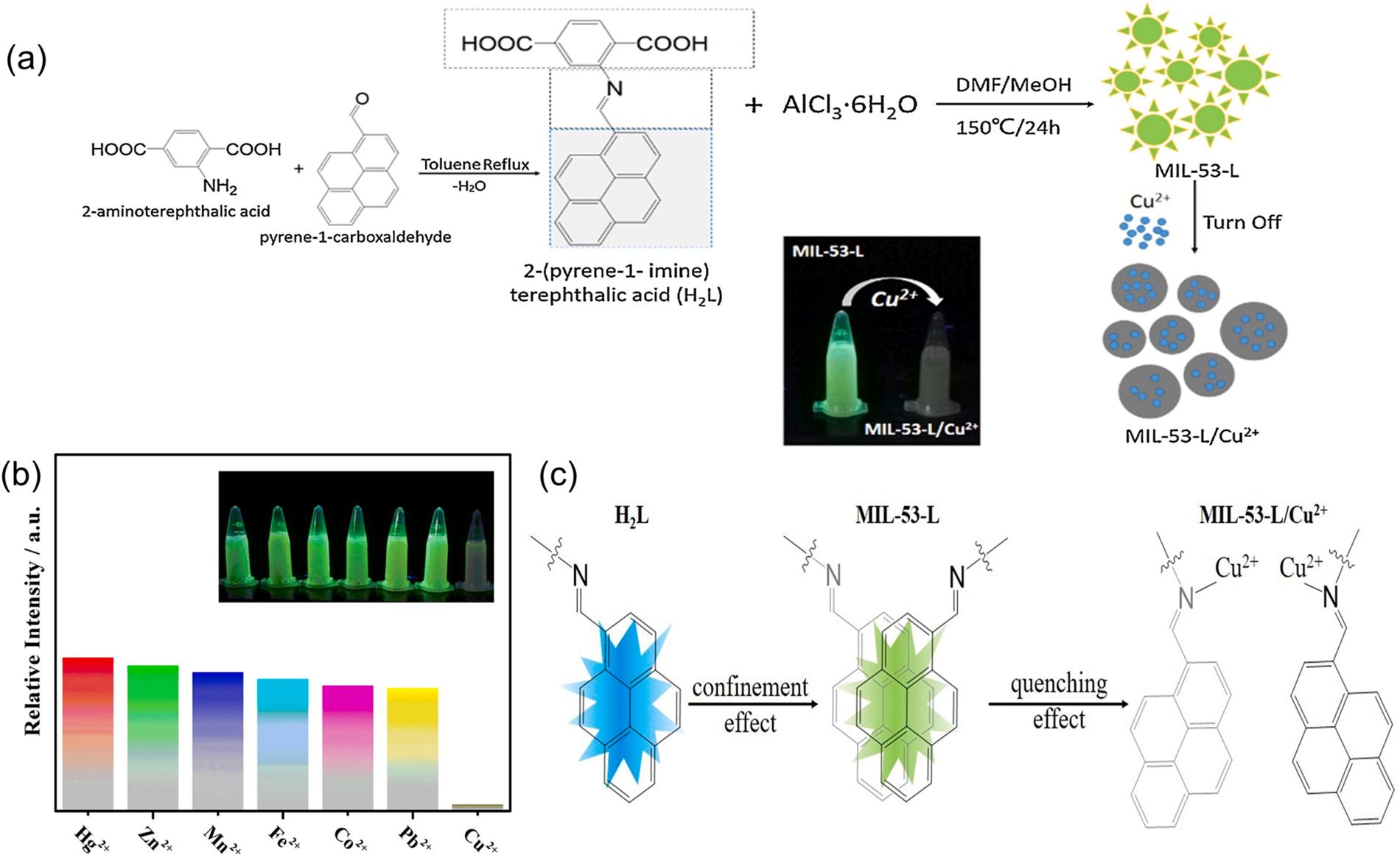
Fig. 10. The application of MIL-53-L for Cu2+ sensing: (a) schematic of MIL-53-L for sensing Cu2+ ions, (b) the relative intensities of MIL-53-L in different metal ions aqueous solution and (c) the possible conformational changes of MIL-53-L for detecting Cu2+ ions (Liu and Yan, 2016), copyright 2016, Elsevier publishing group.
by the intramolecular proton transfer mechanism. Such a mechanism is ascribed to the coordination of Bi3+ ions and hydroxyl groups of CAU-1-(OH)2 through which fluorescence quenching is induced (Gao et al., 2018). It was also found that the fluorescence emission in CAU-1-(OH)2 was quenched by Bi3+ ions in water due to the following possible reasons: (i) parts of Al3+ in the inorganic clusters replaced by Bi3+ caused a change in –COO-Al3+ to –COO-Bi3+ and (ii) Bi3+ ions strongly interacted with the hydroxyl groups of CAU-1-(OH)2, thereby weakening the intramolecular proton transfer process (Fig. 11a). The sensitivity assay demonstrated a linear relationship between the fluorescence emission (505 nm) in CAU-1-(OH)2 and the concentration of (0–1 ×10 4 M) Bi3+ ions under 365 nm excitation (Fig. 11b). The corresponding LOD for the fluorescence quenching assay was determined to be 2.16 µM, highlighting its great potential for real-world applications (Gao et al., 2018).
The luminescent emission in MOFs can be further enhanced by the sensitization process (also called the antenna effect) (Yan, 2017). Specifically, analytes acting as strong photon absorbers transferred the
absorbed energy to the luminescent probe (e.g., luminescent MOFs) as an effective option to enhance the emission intensity of luminescent probes (Lustig et al., 2017). As an example, a lanthanide ion (Ln3+)@Al (OH) (H2btec) H2O (Ln3+@MIL-121) fluorescent probe was designed through the post-modification method (for Ag+ detection) (Hao and Yan, 2015). After post-synthetic functionalization of Ln3+ (e.g., Sm3+), Ag+/Sm3+@MIL-121 showed intense characteristic peaks of Sm3+ due to the antenna effect of Ag+ ions. A typical energy transfer of Ag+/Sm3+@MIL-121 was found as follows. First, the lanthanide-organic ligand complex was excited from the singlet S0 ground state to the singlet S1 excited state upon absorbing the energy. Then, the energy of the S1 excited state was transferred to the triplet T1 excited state of the ligands. Further, the energy of triplet T1 excited state was transferred to the excited f states of the Ln3+ ions (e.g., Sm3+). Finally, the luminescent emission (f-f) of lanthanide Sm3+ ions was generated (Hao and Yan, 2015; Feng and Zhang, 2013)(Fig. 12a). During the above energy process, the antenna effect of Ag+ ions functioned as follows: (i) the Ag+ ions reduced the rate constant for non-radiative deactivation and energy
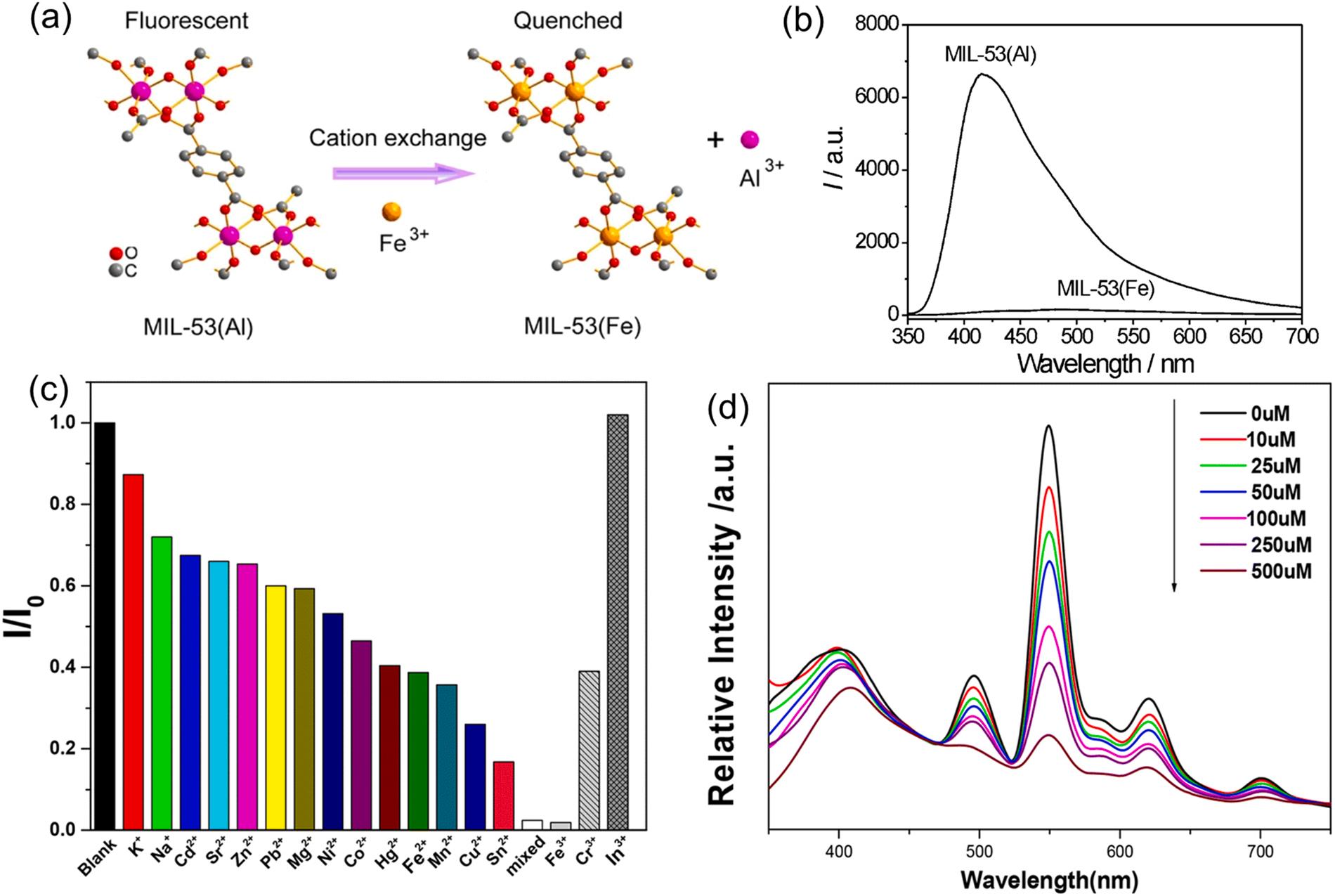
Fig. 11. The application of Al-MOF for Fe3+ ion sensing based on cation exchange. The application of MIL-53(Al) for detection of Fe3+ ions: (a) schematic of the cation exchange mechanism for MIL-53(Al) to sense Fe3+ , (b) fluorescence spectra of MIL-53(Al) and MIL-53(Fe) (Yang et al., 2013), copyright 2013, ACS publishing group. The application of MIL-110(Al)/Tb3+ for detection of Fe3+ ions: (c) luminescent intensity of MIL-110(Al)/Tb3+ against various metal ions in the aqueous solution. (d) The relative intensity of MIL-110(Al)/Tb3+ as a function of Fe3+ ions concentration (Dao and Ni, 2017), copyright 2017, RSC publishing group.
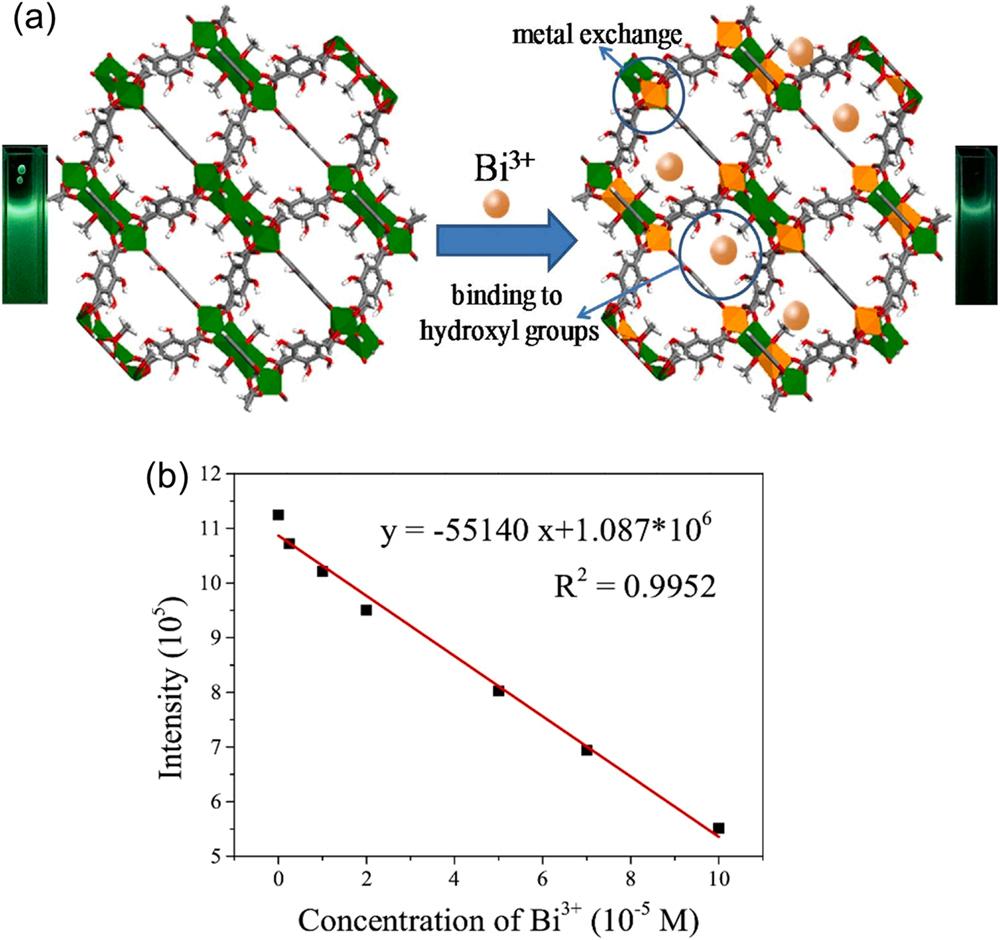
Fig. 12. The application of CAU-1-(OH)2 for Bi3+ ion sensing: (a) the mechanism of CAU-1-(OH)2 for the detection of Bi3+ (green: Al; orange: Bi; gray: C; white: H; red: O) and (b) linear fitting results of fluorescence versus the concentration of Bi3+ ions (Gao et al., 2018), copyright 2018, Elsevier publishing group.
loss of lanthanide complexes during the S0→S1 energy process. This was confirmed by Ag+/Sm3+@MIL-121 having a longer lifetime (97.6 µs) than those of Sm3+@MIL-121 (18.0 µs). (ii) The perturbation of the spin-orbit coupling in Ag+ ions (heavy-atom effect) promoted intersystem crossing of ligand S1→T1 energy transfer (Liu et al., 2012; Pellow and Vala, 1989). (iii) The outer-shell d electrons of Ag+ caused metal-to-ligand charge transfer, which resulted in the sensitization of fluorescent Sm3+ As such, Sm3+@MIL-121 exhibited excellent selectivity for Ag+ as compared to other metal ions (e.g., Na+ , K+ , and Pb2+) (Fig. 12b). All three processes described above helped Ag+ ions contribute to the effective energy transfer from ligands to lanthanide ions, thereby leading to the fluorescent enhancement of Ag+/Sm3+@MIL-121. Furthermore, the Sm3+@MIL-121 exhibited a fast detection time (< 1 min) for the detection of Ag+ ions since the Ag+-induced fluorescence reaction was a rapid process (Fig. 12c). The fluorescence intensity at 603 nm emission of Sm3+@MIL-121 also showed an increase in the linear relationship with increasing Ag+ concentrations (0–500 µM) under excitation at 320 nm (Fig. 12d) (Hao and Yan, 2015).
Recently, electrochemiluminescence (ECL) was found to be a promising method for metal ion sensing due to its advantages including low background and rapid response (Feng et al., 2020). ECL involves the generation of electroactive species through electrochemical reaction at an electrode surface. Such active species undergo high-energy electro-transfer reactions (i.e., reaching excited state) to emit light (Hua et al., 2022). In this regard, sensitive ECL aptasensors for multiple determination of Hg2+ and Pb2+ were designed using MIL-53(Al)@CdTe-PEI and Au NP/Pt NP labelled aptamers as ECL signal probes and target recognition probes, respectively (Fig. 13a) (Feng et al., 2020). The occurrence of enhanced resonance energy transfer was confirmed by the
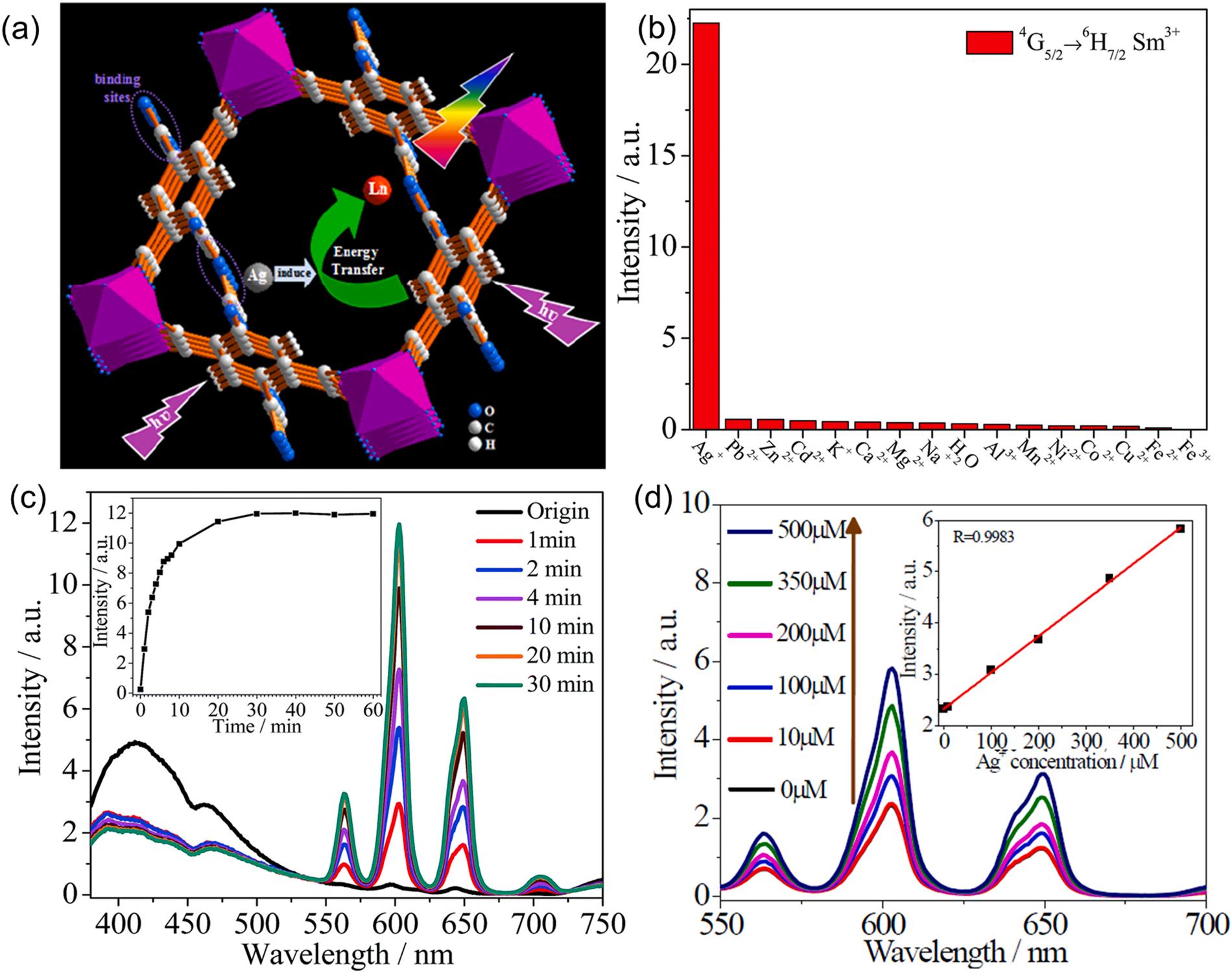
Fig. 13. The application of Sm3+@MIL-121 for sensing Ag+ ions: (a) schematic of the fluorescent enhancement of Sm3+@MIL-121 by Ag+ ions. (b) Relative fluorescence intensities of Sm3+@MIL-121 against various metal ions. (c) Variation of fluorescent intensity of Sm3+@MIL-121 at various immersion times. (d) Fluorescence intensity of Sm3+@MIL-121 as a function of Ag+ ion concentration (Hao and Yan, 2015), copyright 2015, RSC publishing group.
overlap in the emission spectra of the CdTe quantum dots (QDs) and absorption spectra of Au or Pt NPs (Fig. 13b). In addition, the MIL-53(Al) and CdTe significantly enhanced the current flow in the sensor for sensing metal ions due to their high electrical conductivity. As for the Hg2+ ions detection (path 1), the Hg2+ ions were initially recognized by T-rich oligonucleotides (aptamer 2-Au NPs) (Fig. 13a). Then, aptamer 2-Au NPs fell off the ECL electrode after the formation of hairpin DNA structures (T-Hg2+-T base pair). As a result, the dual surface plasmon resonance effect of Au and Pt NPs was replaced with the single surface plasmon effect of Pt NPs to reduce the ECL signal (Eq. 5). The detection of Pb2+ ions was accompanied by the formation of Pb2+-G-quadruplexes (path 2 and 3). As such, the Pt NPs were effectively stacked on the electrode, thereby quenching the ECL intensity of the ECL aptasensor through enhanced resonance energy transfer between Pt NPs and CdTe. In other words, the original energy needed for the excitation of CdTe* was dissipated by Pt NPs as CdTe* emitted fewer photons. The corresponding ECL processes can be listed as follows:
CdTe + e→ CdTe-• (2) S2O82- + e -
53(Al)@CdTe-PEI: 5 µL, scan rate: 100 mV s 1 , aptamer 1-Pt NPs: 14 µL, aptamer 2-Au NPs: 8 µL) (Fig. 14c) (Feng et al., 2020). More recently, a Cu2+/Pb2+ ions electrochemical sensor composed of NH2-MIL-53 (Al)/polypyrrole (PPy) composites was designed (Wang et al., 2020b). NH2-MIL-53(Al) and polypyrrole (PPy) composites were formed through π-π interactions between the PPy and NH2-MIL-53(Al). Such strong interactions gave the NH2-MIL-53(Al)/PPy sensor good stability (90% of initial response remained after 2 weeks). As a result of the synergistic effects between NH2-MIL-53(Al) and PPy, the NH2-MIL-53(Al)/PPy sensor exhibited suitable sensing performance toward Cu2+ and Pb2+ Furthermore, the NH2-MIL-53(Al)/PPy sensor recorded high selectivity towards Cu2+ and Pb2+ ions in the presence of interferent ions (e.g., Cd2+ , Ca2+ , and Zn2+), which can be attributed to the high affinity of amino and carboxyl groups of NH2-MIL-53(Al) for Cu2+/Pb2+ ions (Wang et al., 2020b). Despite the good performance (e.g., low detection limit) of electrochemiluminescence or electrochemical based Al-MOFs, only a few studies have been carried out to assess their sensing capacity toward metal ions as listed in Table 3 (Feng et al., 2020; Wang et al., 2020b).
4. Performance evaluation of Al-MOF-based sensing systems
4.1. Overall performance evaluation of Al-MOF-based sensors
MIL-53(Al)@CdTe-PEI based ECL sensor was used to detect metal ions with a dynamic concentration range under optimal conditions (MIL-
The sensing performances (e.g., in terms of LOD, dynamic detection range, and selectivity) of various Al-MOF based sensors against various
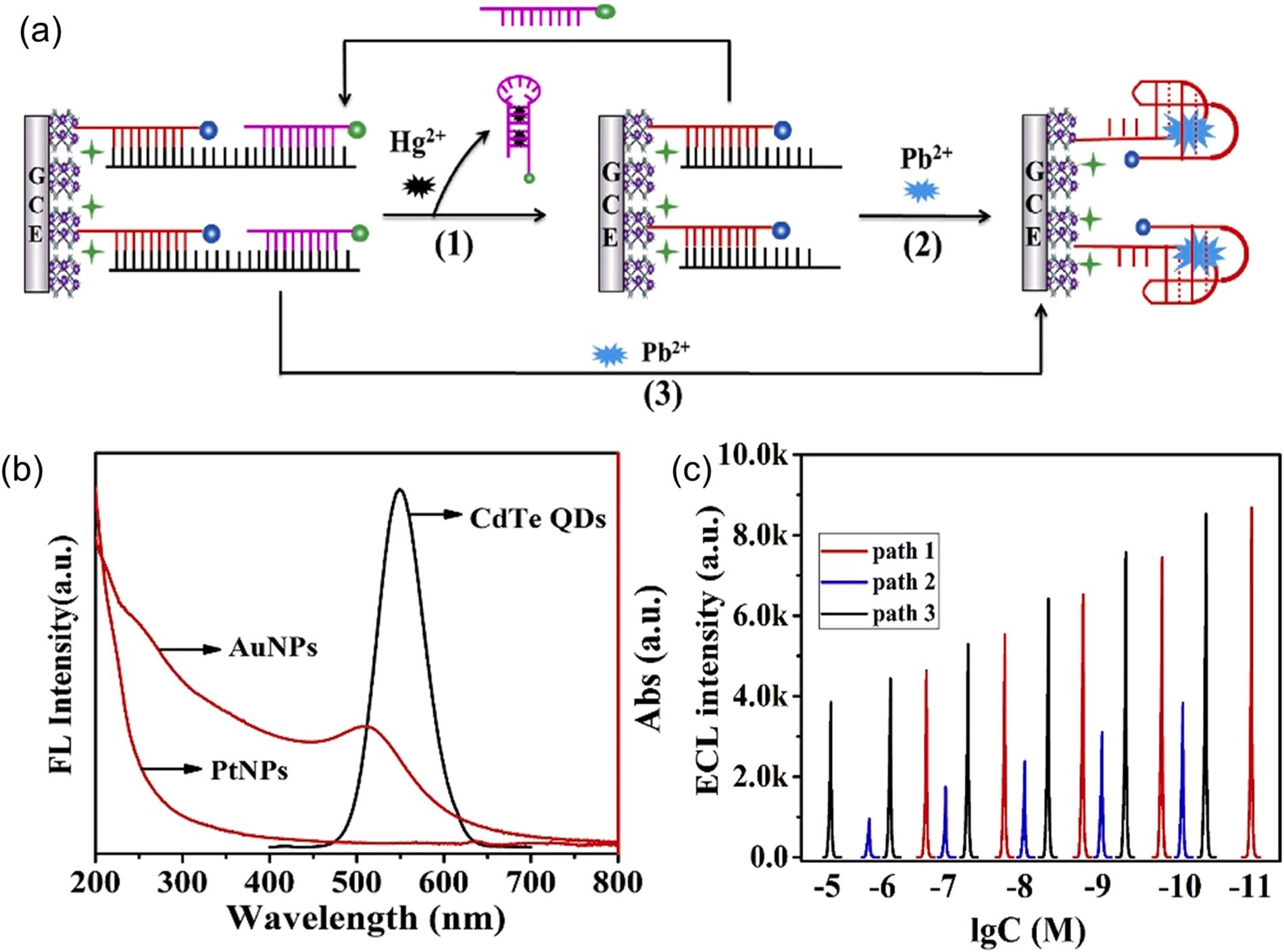
Fig. 14. The application of MIL-53(Al)@CdTe-PEI based ECL sensor for Pb2+/Hg2+ ion detection: (a) schematic of MIL-53(Al)@CdTe-PEI based ECL sensor for Pb2+/Hg2+ ions detection (1: path 1, 2: path 2, and 3: path 3). (b) UV–vis spectra of Pt NPs, Au NPs, and CdTe, (c) ECL emission measured at different concentrations of Hg2+ (path 1) and Pb2+ (path 2 and 3) (Feng et al., 2020), copyright 2020, Elsevier publishing group.
metal ions are summarized in Table 3 In this section, we compare the performance of different types of Al-MOF-based sensors against various metal ions (e.g., Cu2+ , Hg2+ , and Pb2+ ions) mainly in terms of LOD as a key quality assurance metric. In this regard, the top five performers have been selected in terms of LOD, regardless of the metal species and/or sensing principle. Then, the best sensing systems are also selected and discussed for each metal species based on the same criteria (i.e., LOD). Accordingly, the top five performers for the detection of metal ions, if organized in terms of LOD value, were: MIL-53(Al)@CdTe-PEI (Hg2+ , 4.1 ×10 6 µM) > MIL-53(Al)@CdTe-PEI (Pb2+ , 2.4 ×10 5 µM) > NH2MIL-53(Al) (Hg2+ , 0.23 ×10 3 µM) > NH2-MIL-53(Al)/Ppy (Cu2+ , 3.84 ×10 3 µM) (Feng et al., 2020; Wang et al., 2020b; Ren et al., 2020). Here, the highest sensing ability of the MIL-53(Al)@CdTe-PEI-based electrochemiluminescent sensor against Hg2+ was attributed to the sensitive and specific recognition element (aptamer 2) toward Hg2+ ions. Besides, the utilization of CdTe quantum dots endowed the Hg2+ ion sensor with strong ECL emission due to its good electrical conductivity and high quantum yield. The high specific surface area of MIL-53 (Al) acted as good supporter for CdTe quantum dots (Feng et al., 2020). Besides, such MIL-53(Al)@CdTe-PEI-based electrochemiluminescent sensors also provided the best sensing performance (2.4 ×10 5 µM) toward Pb2+ ions in an aqueous solution when using aptamer 1 as a recognition element (Feng et al., 2020). The sensing performance of Al-MOF-based electrochemiluminescent sensors was generally noticeable in the detection of divalent metal ions (e.g., Pb2+ and Hg2+) over other Al-MOF-based sensors (e.g., electrochemical and luminescent sensors) (Table 3). Such results might reflect the highly sensitive characteristics of electrochemiluminescent techniques using the low
background noise (Li et al., 2017). Interestingly, luminescent-based Al-MOFs were widely reported for the detection of metal ions over other types of sensors (e.g., electrochemical and electrochemiluminescence) (Table 3). The good universality of luminescent-based Al-MOFs might be attributed to their simple formulations, ease of operation, and cost-effectiveness.
The best performers in terms of lowest LOD value for each metal species were selected for evaluation. In the case of Fe3+ ions, the Al-MIL53-N3-based luminescent sensor was found to have the best LOD (0.03 µM) (Das et al., 2018). Its superior performance may be attributed to two factors including: (i) the interaction of electron-withdrawing –N3 groups of 2-azido-1,4-benzenedicarboxylic acid ligands with electron-deficient Fe3+ ions and (ii) partial cation exchange between Al3+ and Fe3+ ions during the sensing process. The synergic effect of both processes endows the Al-MIL-53-N3-based luminescent sensor with the highly sensitive detection of Fe3+ ions in aqueous media (Das et al., 2018). In addition, the aluminum CAU-1-(OH)2-based luminescent sensor showed the best Bi3+ ion sensing performance with a LOD value (2.16 µM) (Gao et al., 2018). Here, the carboxylate and hydroxyl groups in CAU-1-(OH)2 can strongly coordinate with Bi3+ ions. The high specific surface area (1386 m2 g 1) of CAU-1-(OH)2 offered a large number of active sites for sensing Bi3+ ions (Gao et al., 2018). Likewise, because of the large pore properties of MOF-253, Fe2+ ions can easily enter into the voids to react with the bipyridine group of MOF-253 (Lu et al., 2014). As such, the aluminum MOF-253-based luminescent sensor offered the best sensitivity (LOD: 0.5 µM) for detecting Fe2+ ions (Lu et al., 2014).
The best Cu2+ ion sensing system was identified as the NH2-MIL-53
(Al)/Ppy-based electrochemical sensor (LOD: 3.84 ×10 3 µM) (Wang et al., 2020b). The performance of this Cu2+ ion sensing platform reflected the synergy between high specific area of the NH2-MIL-53(Al) and the excellent conductivity of the PPy nanowires that boosted active sites for the detection of Cu2+ ions (Wang et al., 2020b). Further, the nitrogen on the pyrrole unit of PPy nanowires can effectively coordinate with Cu2+ ions. The amino and carboxylic groups from NH2-MIL-53(Al) had metal ions chelation properties for Cu2+ ions. As such, NH2-MIL-53 (Al)/Ppy-based electrochemical sensors can efficiently detect Cu2+ ions at the lowest LOD level of 3.84 × 10 3 µM based on the differential pulse voltammetry (DPV) technique (Wang et al., 2020b). In addition, the best Ag+ sensing system with a low LOD value of 0.09 µM was achieved using a Sm3+@MIL-121-based luminescent sensor due to the antenna effect of
Table 4
Ag+ ions (Hao and Yan, 2015). Such an antenna effect enhanced the energy transfer from ligands to Sm3+ . As such, the Sm3+@MIL-121-based luminescent sensor can be highly sensitive (LOD: 0.09 µM) for detecting Ag+ ions (Hao and Yan, 2015). In the case of Pd2+ ion detection, the CAU-10-V-H-based luminescent sensor showcased the lowest LOD value (0.11 µM) among all Pd2+ sensing systems (Nandi et al., 2020). Such superior performance of the CAU-10-V-H-based luminescent sensor should be ascribable to the strong interactions between Pd2+ ions and vinyl functional groups of 5-vinyl isophthalic acid (H2IPA-V) from CAU-10-V-H (Nandi et al., 2020). The cyclic utilization of this sensor was also examined up to five cycles after thorough washing in each experimental cycle. Accordingly, about 70% of reduction in the sensing quenching efficiency occurred after five cycles due to the
Comparison between Al-MOF-based sensor and non-Al-MOF-based sensor for sensing various metal ions.
No. Sensors Sensing
[A] Fe3þ ions
1 Al-MIL-53-N3-based luminescent sensor Water 0.03 – (Das et al., 2018)
2 Eu-MOF-based luminescent sensor
(Guo et al., 2020)
3 [In(L)(m2-OH)]0.5 H2O-based luminescent sensor Aqueous solution 21 – (Cen et al., 2020)
4 Cd-MOF-based luminescent sensor Aqueous solution
0–16 (Lv et al., 2018)
5 [ZnL] 2 H2O-based luminescent sensor Aqueous solution 0.92 1–1000 (Li et al., 2018) [B] Bi3þ ions
6 CAU-1-(OH)2-based luminescent sensor Aqueous solution 2.16 0–100 (Gao et al., 2018)
7 N-hydroxy 1,8-naphthalimide luminescent sensor
8 Rhodamine 6 G-based luminescent sensor Drugs
– (Ramasamy and Thambusamy, 2017)
10–35 (Zhang et al., 2018a)
9 Glutathione/copper NPs luminescent sensor – 10000 (0–100) × 103 (Wu et al., 2022) [C] Pb2þ ions
10 MIL-53(Al)@CdTe-PEI electrochemiluminescent sensor Aqueous solution 2.4 × 10 5 10 4 - 10 (Feng et al., 2020)
11 Glutathione/thioglycolic acid/CdTe electrochemiluminescent sensor – 0.26 × 10 3 – (Wang et al., 2012)
12 CdS QDs electrochemiluminescent sensor
13 CdS QDs/graphene/gold electrochemiluminescent sensor
14 CdSe/ZnS electrochemiluminescent sensor Aqueous solution
3.74 × 10 5 (0.2–50) × 10 3 (Xiao-Ping et al., 2014)
1 × 10 4 (0.1–10) × 10 3 (Lu et al., 2016)
× 10 3 (0–76) × 10 3 (Wang et al., 2015b) [D] Pd2þ ions
15 CAU-10-V-H-based luminescent sensor Water 0.11 – (Nandi et al., 2020)
16 {[Zn-(ATA)(L)] H2O}n-based luminescent sensor Water 0.2 0–25 (Parmar et al., 2017)
17 3-(1-isoquinolinyl)imidazo[5, 1-a]isoquinoline luminescent sensor Water
– (Mahata et al., 2020)
18 Quinoline-benzimidazole conjugate material luminescent sensor Water 0.26 0.5–10 (Wang et al., 2021) [E] Hg2þ ions
19 MIL-53(Al)@CdTe-PEI electrochemiluminescent sensor Aqueous solution 4.1 × 10 6 10 5 - 10 1 (Feng et al., 2020)
20 Fe-single atom catalysts electrochemiluminescent sensor Aqueous solution
21 Polyluminol gold nanocomposite electrochemiluminescent sensor Water
22 Gold NP-modified indium tin oxide electrochemiluminescent sensor Water
23 Micro fluidic paper-based electrochemiluminescent sensor Water
× 10 3 (0.01 0.5) × 10 3 (Bushira et al., 2021)
× 10 3 (0.3–200) × 10 3 (Raju and Kumar, 2021)
× 10 6 (0.02–30) × 10 3 (Huang et al., 2015)
× 10 3 0.0005–1 (Zhang et al., 2013) [F] Cu2+ ions
24 NH2-MIL-53(Al)/Ppy-based electrochemical sensor Aqueous solution
25 NH2-Ni-MOF-baed electrochemical sensor Water
26 Graphene aerogel/UiO-66-NH2-based electrochemical sensor Water
× 10 3 0.015–6.294 (Wang et al., 2020b)
× 10 3 0.01–2 (Wan et al., 2021)
× 10 3 0.1–3.5 (Lu et al., 2018)
27 Cu3(HHTP)2-based electrochemical sensor Aqueous solution 0.331 100–
28 COFBTLP-based electrochemical sensor
29 Covalent-organic framework-based electrochemical sensor Water
(Xu et al., 2022)
–18 (Han et al., 2020)
× 10 3 0.0034–4 (Pei et al., 2022) [G] Fe2+ ions
30 MOF-253-based luminescent sensor Aqueous solution
31 [Eu2(IMBA)6(dmp)2]-based luminescent sensor
32 Ni-MOF based luminescent sensor Aqueous solution
33 Triphenylamine/2,2′ -bipyridine-5,5′ -diformaldehyde based luminescent sensor Water
34 Sicyanomethylene-4 H-pyran based luminescent sensor Living cells
5–
–
(Lu et al., 2014)
(Zhao et al., 2018)
– (Asiwal et al., 2022)
(Zhang et al., 2022b)
0–100 (Yang et al., 2019) [H] Agþ ions
35 Sm3þ@MIL-121-based luminescent sensor Aqueous solution
36 Carbon dot luminescent sensor Aqueous solution
0–
(Hao and Yan, 2015)
(0–2.66)× 103 (Algarra et al., 2014)
37 [ZnIJBTA)2]n luminescent sensor Aqueous solution 9 – (Shao et al., 2022)
38 Bis-heteroleptic Ru(II) complex luminescent sensor Carbonate-bicarbonate buffer/ CH3CN solution
39 {[Zn6Cl6(2,2′-dbpt)3] 4.5 H2O}n luminescent sensor Aqueous solution
0–250 (Sen et al., 2021)
(Cao et al., 2020)
complex formation between Pd2+ ions and vinyl functionality of CAU-10-V-H materials (Nandi et al., 2020). More efforts are thus needed to improve its practicality.
4.2. Comparing the performance of Al-MOF-based sensors with other common sensors
The performance metrics (e.g., LOD and dynamic detection range) of Al-MOF-based sensors were also compared with other common sensors including MOF-based sensors and non-MOF-based sensors to learn more about the uses of Al-MOF-based sensors in the detection of various metal ions (Table 4). For this purpose, the best Al-MOF-based sensing systems were compared with the best non-Al-MOF-based sensing systems for detecting various metal ions (e.g., Ag+ , Pd2+ , and Fe3+). As summarized in Table 4, the aluminum CAU-1-(OH)2-based luminescent sensor (with LOD value of 2.16 µM), having a wide linear detection range (0–100 µM), outperformed the non-Al-MOF-based sensor (e.g., rhodamine 6 G-based luminescent sensor, LOD: 2.69 µM, linear detection range: 10–35 µM) for the detection of Bi3+ ions (Gao et al., 2018; Zhang et al., 2018a). In case of Fe3+ detection, an Al-MIL-53-N3-based luminescent sensor with an LOD value of 0.03 µM exhibited a higher sensing ability than Cd-MOF-based luminescent sensor (LOD = 0.3 µM) (Das et al., 2018; Lv et al., 2018). In addition, the Al-MOF-253-based luminescent sensor exhibited significantly higher sensitivity for detecting Fe2+ ions (0.5 µM) than a [Eu2(IMBA)6(dmp)2] MOF-based luminescent sensor (1 µM) (Lu et al., 2014; Zhao et al., 2018). For the detection of Pd2+ , the Al-CAU-10-V-H-based luminescent sensor offered a low LOD value (0.11 µM) as compared to a non-Al-based zinc {[Zn-(ATA)(L)]⋅ H2O}n luminescent sensor (0.2 µM) (Nandi et al., 2020; Parmar et al., 2017). The detection limit of MIL-53(Al)@CdTe-PEI-based electrochemiluminescent sensor was noticeably lower for Pb2+ ions (2.4 ×10 5 µM) than that of a CdS QDs electrochemiluminescent sensor (3.74 ×10 5 µM) (Feng et al., 2020; Xiao-Ping et al., 2014). Regarding the detection of monovalent ions (e.g., Ag+), a Sm3+@MIL-121-based luminescent sensor showed a superior LOD value (0.09 µM) compared with that of a carbon dot luminescent sensor (0.386 µM) (Hao and Yan, 2015; Algarra et al., 2014). Nevertheless, in the Cu2+ sensing systems, the covalent-organic framework (COF)-based electrochemical sensor (i. e., non-Al-MOF-based sensor) exhibited better sensitivity (LOD: 1.14 ×10 3 µM) than those of NH2-MIL-53(Al)/Ppy-based electrochemical sensors (LOD: 3.84 ×10 3 µM) (Wang et al., 2020b; Pei et al., 2022). The better performance of a COF-based electrochemical sensor may be attributed to the abundant actives sites (e.g., N-S-N and –C–– N) for capturing Cu2+ ions in the highly ordered COF-based conjugated structure (Pei et al., 2022).
In light of these above findings, the sensing performance of Al-MOFbased sensors showed a better performance in terms of lowest LOD value compared with other common MOF-based and non-MOF based sensors for various metal ions (e.g., Pb2+ , Ag+ , Bi3+ , Pd2+ , Fe3+ , and Fe2+) as listed in Table 4 Moreover, the LOD of MIL-53(Al)@CdTe-PEI-based electrochemiluminescent sensor was moderately superior in metal ions detection (Pb2+: 2.4 ×10 5 µM) to some reported classical techniques including ICP-OES (LOD: 4.83 ×10 5 µM) and ICP-MS (LOD: 2.89 ×10 5 µM) (Feng et al., 2020; Hosseinzadegan et al., 2019; Voica et al., 2009). As such, it is revealed that Al-MOF-based sensors can be regarded as a promising option for the detection of various metal ions in aqueous system.
5. Conclusion and outlook
Al-MOFs can overcome many disadvantages of common MOFs (e.g., poor thermal, chemical, and water stability) to allow the expansion of their applications toward various fields (e.g., detection of metals in aqueous medium). In light of the beneficial properties of Al-MOFs, this comprehensive review is offered to describe the latest progress achieved in their synthesis (e.g., solvo/hydrothermal, reflux method,
sonochemical, microwave-assisted method, electrochemical, and mechanochemical method) in relation to their merits and disadvantages. The architectural versatility of Al-MOFs enables their facile functionalization with advanced sensing elements (e.g., fluorescent organic ligands, lanthanide ions, and CdTe quantum dots). As such, the functionalized Al-MOFs have become a favorable medium for the detection of metal ions in the aqueous system using (i) fluorescence intensity, (ii) quantum yield, (iii) conductivity, and (iv) electric catalytic activity. The analytical performance of various Al-MOF-based sensors used for the detection of metal ions has been discussed to ensure their superior sensing performance (in terms of detection limit) over non-AlMOF-based sensors. However, some roadblocks still need to be addressed in real-world applications as described below:
(1) Extensive efforts should be put forward to guarantee the feasibility of cyclic utilization of Al-MOF-based metal ion for their practical applications in the sensing fields. Moreover, the utilization of some novel techniques (e.g., MOFs test paper) could be considered as an effective tool to avoid possible lost issue of powder state MOFs during application. As such, more efficient strategies should be proposed in the near future research.
(2) More efforts should be made to explore continuous flow synthesis routes for maintaining the high quality (e.g., crystallinity and narrow size distribution) of the fabricated Al-MOFs products. In addition, continuous synthesis routes can be used to overcome the shortcomings of the batch process (e.g., low efficiency and lack of flexibility). As such, a continuous flow synthesis system can be employed to scale up their industrial applications.
(3) The introduction of functional materials with a variety of properties (e.g., excellent conductivity) into Al-MOFs can endow the Al-MOF composites with upgraded properties for sensing applications. As such, Al-MOF composites can make use of various sensitive signal transduction principles (e.g., electrochemical) for highly sensitive and selective detection of analytes.
(4) Efforts should be put to upscale from the laboratory research into industrial applications. To date, the potential of Al-MOF-based sensors has mainly been explored at lab-scale. Thus, it is indispensable to find a large-scale synthesis route to expand the realworld applications of Al-MOF-based sensors.
Environmental implication
Toxic heavy metals in the environment are responsible for the various diseases and disasters. They cause many environmental problems. Therefore, their detection from environment is very important. Chemical sensing detection of metal ions from water is regarded as high sensitivity and facile technology. Al-MOFs have received considerable attention due to their merits of low cost, good water stability and thermal stability. Al-MOFs sensing system can be formulated via direct synthesis, post-synthetic modification, and composite material preparation. Such formulation can efficiently enhance the interactions between the Al-MOFs and target heavy metal ions to promote the selective detection of heavy metal ions. The development of Al-MOFs for highly detection of heavy metal ions in liquid phase with respect to their performance and associated detection mechanisms.
Declaration of Competing Interest
The authors declare that they have no known competing financial interests or personal relationships that could have appeared to influence the work reported in this paper.
Data Availability
Data will be made available on request.
Acknowledgments
This work was supported partially by a grant from the National Research Foundation of Korea (NRF) funded by the Ministry of Science and ITC (MSIT) of the Korean government (Grant No: 2021R1A3B1068304).
References
Aguilera-Sigalat, J., Bradshaw, D., 2016. Synthesis and applications of metal-organic framework–quantum dot (QD@ MOF) composites. Coord. Chem. Rev. 307, 267–291
Aguirre-Díaz, L.M., Gandara, F., Iglesias, M., Snejko, N., Gutierrez-Puebla, E., Monge, M. ´ A., 2015. Tunable catalytic activity of solid solution metal–organic frameworks in one-pot multicomponent reactions. J. Am. Chem. Soc. 137, 6132–6135
Aguirre-Díaz, L.M., Reinares-Fisac, D., Iglesias, M., Gutierrez-Puebla, E., Gandara, F., Snejko, N., Monge, M. ´ A., 2017. Group 13th metal-organic frameworks and their role in heterogeneous catalysis. Coord. Chem. Rev. 335, 1–27
Ahadi, N., Askari, S., Fouladitajar, A., Akbari, I., 2022. Facile synthesis of hierarchically structured MIL-53 (Al) with superior properties using an environmentally-friendly ultrasonic method for separating lead ions from aqueous solutions. Sci. Rep. 12, 1–17
Ahmed, S., Khan, F.S.A., Mubarak, N.M., Khalid, M., Tan, Y.H., Mazari, S.A., Karri, R.R., Abdullah, E.C., 2021. Emerging pollutants and their removal using visible-light responsive photocatalysis–a comprehensive review. J. Environ. Chem. Eng. 9, 106643
Ahnfeldt, T., Stock, N., 2012. Synthesis of isoreticular CAU-1 compounds: effects of linker and heating methods on the kinetics of the synthesis. CrystEngComm 14, 505–511.
Ahnfeldt, T., Guillou, N., Gunzelmann, D., Margiolaki, I., Loiseau, T., F´ erey, G., Senker, J., Stock, N., 2009. [Al4 (OH) 2 (OCH3) 4 (H2N-bdc) 3]⋅ x H2O: a 12–connected porous metal–organic framework with an unprecedented aluminumcontaining brick. Angew. Chem. Int. Ed. 48, 5163–5166
Ahnfeldt, T., Moellmer, J., Guillerm, V., Staudt, R., Serre, C., Stock, N., 2011. Highthroughput and time-resolved energy-dispersive X-ray diffraction (EDXRD) study of the formation of CAU-1–(OH) 2: microwave and conventional heating. Chem. Eur. J. 17, 6462–6468
Al-Attri, R., Halladj, R., Askari, S., 2022. Green route of flexible Al-MOF synthesis with superior properties at low energy consumption assisted by ultrasound waves. Solid State Sci. 123, 106782
Algarra, M., Campos, B.B., Radotic, K., Mutavdzic, D., Bandosz, T., Jimenez-Jimenez, J., Rodriguez-Castellon, E., da Silva, J.C.E., 2014. Luminescent carbon nanoparticles: effects of chemical functionalization, and evaluation of Ag+ sensing properties. J. Mater. Chem. A 2, 8342–8351
Asiwal, E.P., Shelar, D.S., Manjare, S.T., Pawar, S.D., 2022. Ni-MOF based luminescent sensor for selective and rapid sensing of Fe (II) and Fe (III) ions. New J. Chem. Banica, F.-G., 2012. Chemical Sensors and Biosensors: Fundamentals and Applications. John Wiley & Sons
Barth, B., Mendt, M., Poppl, A., Hartmann, M., 2015. Adsorption of nitric oxide in metalorganic frameworks: Low temperature IR and EPR spectroscopic evaluation of the role of open metal sites. Microporous Mesoporous Mater. 216, 97–110 Bloch, E.D., Britt, D., Lee, C., Doonan, C.J., Uribe-Romo, F.J., Furukawa, H., Long, J.R., Yaghi, O.M., 2010. Metal insertion in a microporous metal organic framework lined with 2, 2′ -bipyridine. J. Am. Chem. Soc. 132, 14382–14384.
Bushira, F.A., Kitte, S.A., Xu, C., Li, H., Zheng, L., Wang, P., Jin, Y., 2021. Twodimensional-plasmon-boosted iron single-atom electrochemiluminescence for the ultrasensitive detection of dopamine, hemin, and mercury. Anal. Chem. 93, 9949–9957
Cadiau, A., Lee, J.S., Damasceno Borges, D., Fabry, P., Devic, T., Wharmby, M.T., Martineau, C., Foucher, D., Taulelle, F., Jun, C.H., 2015. Design of hydrophilic metal organic framework water adsorbents for heat reallocation. Adv. Mater. 27, 4775–4780
Camilli, L., Passacantando, M., 2018. Advances on sensors based on carbon nanotubes. Chemosensors 6, 62
Cao, Y., Zhang, Y., Gu, L.-W., Qin, X.-M., Li, H.-Y., Bian, H.-D., Huang, F.-P., 2020. A zinc 2+-dpbt framework: luminescence sensing of Cu 2+, Ag+, MnO 4 and Cr (VI)(Cr 2 O 7 2 and CrO 4 2 ) ions. N. J. Chem. 44, 10681–10688
Cen, P., Liang, C., Duan, L., Wang, M., Tian, D., Liu, X., 2020. A robust 3D In–MOF with an imidazole acid ligand as a fluorescent sensor for sensitive and selective detection of Fe 3+ ions. N. J. Chem. 44, 16076–16081
Chen, D., Zhao, J., Zhang, P., Dai, S., 2019b. Mechanochemical synthesis of metal–organic frameworks. Polyhedron 162, 59–64
Chen, L., Liu, D., Peng, J., Du, Q., He, H., 2020. Ratiometric fluorescence sensing of metal-organic frameworks: tactics and perspectives. Coord. Chem. Rev. 404, 213113
Chen, Z., Hanna, S.L., Redfern, L.R., Alezi, D., Islamoglu, T., Farha, O.K., 2019a. Reticular chemistry in the rational synthesis of functional zirconium cluster-based MOFs. Coord. Chem. Rev. 386, 32–49.
Cheng, X., Zhang, A., Hou, K., Liu, M., Wang, Y., Song, C., Zhang, G., Guo, X., 2013. Sizeand morphology-controlled NH 2-MIL-53 (Al) prepared in DMF–water mixed solvents. Dalton Trans. 42, 13698–13705
Cho, K.H., Borges, D.D., Lee, U., Lee, J.S., Yoon, J.W., Cho, S.J., Park, J., Lombardo, W., Moon, D., Sapienza, A., 2020. Rational design of a robust aluminum metal-organic
framework for multi-purpose water-sorption-driven heat allocations. Nat. Commun. 11, 1–8
Cohen, S.M., 2012. Postsynthetic methods for the functionalization of metal–organic frameworks. Chem. Rev. 112, 970–1000
Comotti, A., Bracco, S., Sozzani, P., Horike, S., Matsuda, R., Chen, J., Takata, M., Kubota, Y., Kitagawa, S., 2008. Nanochannels of two distinct cross-sections in a porous Al-based coordination polymer. J. Am. Chem. Soc. 130, 13664–13672
Crawford, D., Casaban, J., Haydon, R., Giri, N., McNally, T., James, S.L., 2015. Synthesis by extrusion: continuous, large-scale preparation of MOFs using little or no solvent. Chem. Sci. 6, 1645–1649
Crawford, D.E., Casaban, J., 2016. Recent developments in mechanochemical materials synthesis by extrusion. Adv. Mater. 28, 5747–5754
Cui, Y., Yue, Y., Qian, G., Chen, B., 2012. Luminescent functional metal–organic frameworks. Chem. Rev. 112, 1126–1162.
Cui, Y., Chen, B., Qian, G., 2014. Lanthanide metal-organic frameworks for luminescent sensing and light-emitting applications. Coord. Chem. Rev. 273, 76–86
Dao, X., Ni, Y., 2017. Al-Based coordination polymer nanotubes: simple preparation, post-modification and application in Fe 3+ ions sensing. Dalton Trans. 46, 5373–5383
Dao, X., Ni, Y., Pan, H., 2018. MIL-53 (Al)/Eu3+ luminescent nanocrystals: solventadjusted shape-controllable synthesis and highly selective detections for Fe3+ ions, Cr2O72 anions and acetone. Sens. Actuators B Chem. 271, 33–43
Das, A., Banesh, S., Trivedi, V., Biswas, S., 2018. Extraordinary sensitivity for H 2 S and Fe (III) sensing in aqueous medium by Al-MIL-53-N 3 metal–organic framework: In vitro and in vivo applications of H 2 S sensing. Dalton Trans. 47, 2690–2700
Devic, T., Serre, C., 2014. High valence 3p and transition metal based MOFs. Chem. Soc. Rev. 43, 6097–6115
Díaz-Ramírez, M.L., Vargas, B., ´ Alvarez, J.R., Landeros-Rivera, B., Rivera-Almazo, M., Ramos, C., Flores, J.G., Morales, E., Vargas, R., Garza, J., 2020. Fluorometric detection of iodine by MIL-53 (Al)-TDC. Dalton Trans. 49, 6572–6577
Ding, M., Cai, X., Jiang, H.-L., 2019. Improving MOF stability: approaches and applications. Chem. Sci. 10, 10209–10230
Duan, D., Si, X., Ding, Y., Li, L., Ma, G., Zhang, L., Jian, B., 2019. A novel molecularly imprinted electrochemical sensor based on double sensitization by MOF/CNTs and Prussian blue for detection of 17β-estradiol. Bioelectrochemistry 129, 211–217
Fan, Y., Li, C., Zhang, X., Yang, X., Su, X., Ye, H., Li, N., 2019. Troger’s base mixed matrix membranes for gas separation incorporating NH2-MIL-53 (Al) nanocrystals. J. Membr. Sci. 573, 359–369.
Fang, X., Zong, B., Mao, S., 2018. Metal–organic framework-based sensors for environmental contaminant sensing. Nano-Micro Lett. 10, 1–19
Feng, D., Li, P., Tan, X., Wu, Y., Wei, F., Du, F., Ai, C., Luo, Y., Chen, Q., Han, H., 2020. Electrochemiluminescence aptasensor for multiple determination of Hg2+ and Pb2+ ions by using the MIL-53 (Al)@ CdTe-PEI modified electrode. Anal. Chim. Acta 1100, 232–239
Feng, D., Wei, F., Wu, Y., Tan, X., Li, F., Lu, Y., Fan, G., Han, H., 2021. A novel signal amplified electrochemiluminescence biosensor based on MIL-53 (Al)@ CdS QDs and SiO 2@ AuNPs for trichlorfon detection. Analyst 146, 1295–1302
Feng, J., Zhang, H., 2013. Hybrid materials based on lanthanide organic complexes: a review. Chem. Soc. Rev. 42, 387–410
Frohlich, D., Pantatosaki, E., Kolokathis, P.D., Markey, K., Reinsch, H., Baumgartner, M., van der Veen, M.A., De Vos, D.E., Stock, N., Papadopoulos, G.K., 2016. Water adsorption behaviour of CAU-10-H: a thorough investigation of its structure–property relationships. J. Mater. Chem. A 4, 11859–11869
Gaab, M., Trukhan, N., Maurer, S., Gummaraju, R., Müller, U., 2012. The progression of Al-based metal-organic frameworks–From academic research to industrial production and applications. Microporous Mesoporous Mater. 157, 131–136 G´ andara, F., Furukawa, H., Lee, S., Yaghi, O.M., 2014. High methane storage capacity in aluminum metal–organic frameworks. J. Am. Chem. Soc. 136, 5271–5274
Gao, X., Zhao, H., Zhao, X., Li, Z., Gao, Z., Wang, Y., Huang, H., 2018. Aqueous phase sensing of bismuth ion using fluorescent metal-organic framework. Sens. Actuators B Chem. 266, 323–328
García M´ arquez, A., Demessence, A., Platero-Prats, A.E., Heurtaux, D., Horcajada, P., Serre, C., Chang, J.S., F´ erey, G., de la Pena-O’Shea, V.A., Boissi` ere, C., 2012. Green microwave synthesis of MIL-100 (Al, Cr, Fe) nanoparticles for thin-film elaboration. Eur. J. Inorg. Chem. 2012, 5165–5174
Ghoorchian, A., Afkhami, A., Madrakian, T., Ahmadi, M., 2020. Chapter 9electrochemical synthesis of MOFs. In: Mozafari, M. (Ed.), Metal-Organic Frameworks for Biomedical Applications. Woodhead Publishing, pp. 177–195
Ghosh, S., Steinke, F., Rana, A., Alam, M., Biswas, S., Metal-Organic, A., 2021. Framework with allyloxy functionalization for aqueous-phase fluorescence recognition of Pd (II) Ion. Eur. J. Inorg. Chem. 2021, 3846–3851
Guo, H., Wu, N., Xue, R., Liu, H., Li, L., Wang, M.-Y., Yao, W.-Q., Li, Q., Yang, W., 2020. Multifunctional Ln-MOF luminescent probe displaying superior capabilities for highly selective sensing of Fe3+ and Al3+ ions and nitrotoluene. Colloids Surf. A: Physicochem. Eng. Asp. 585, 124094
Guo, Y., Zhang, J., Dong, L.Z., Xu, Y., Han, W., Fang, M., Liu, H.K., Wu, Y., Lan, Y.Q., 2017. Syntheses of exceptionally stable aluminum (III) metal–organic frameworks: how to grow high-quality, large, single crystals. Chem. Eur. J. 23, 15518–15528
Halis, S., Reimer, N., Klinkebiel, A., Lüning, U., Stock, N., 2015. Four new Al-based microporous metal-organic framework compounds with MIL-53-type structure containing functionalized extended linker molecules. Microporous Mesoporous Mater. 216, 13–19
Han, J., Yu, J., Guo, Y., Wang, L., Song, Y., 2020. COFBTLP-1/three-dimensional macroporous carbon electrode for simultaneous electrochemical detection of Cd2+, Pb2+, Cu2+ and Hg2+ Sens. Actuators B: Chem. 321, 128498
Hao, J.-N., Yan, B., 2014. Highly sensitive and selective fluorescent probe for Ag+ based on a Eu 3+ post-functionalized metal–organic framework in aqueous media. J. Mater. Chem. A 2, 18018–18025
Hao, J.-N., Yan, B., 2015. Ag+-sensitized lanthanide luminescence in Ln 3+ postfunctionalized metal–organic frameworks and Ag+ sensing. J. Mater. Chem. A 3, 4788–4792
Hao, Z., Song, X., Zhu, M., Meng, X., Zhao, S., Su, S., Yang, W., Song, S., Zhang, H., 2013. One-dimensional channel-structured Eu-MOF for sensing small organic molecules and Cu 2+ ion. J. Mater. Chem. A 1, 11043–11050
Haque, E., Lo, V., Minett, A.I., Harris, A.T., Church, T.L., 2014. Dichotomous adsorption behaviour of dyes on an amino-functionalised metal–organic framework, aminoMIL-101 (Al). J. Mater. Chem. A 2, 193–203
Henry, B., Samokhvalov, A., 2022. Hygroscopic metal-organic framework MIL-160 (Al): in-situ time-dependent ATR-FTIR and gravimetric study of mechanism and kinetics of water vapor sorption. Spectrochim. Acta Part A Mol. Biomol. Spectrosc. 267, 120550
Hosseinzadegan, S., Nischkauer, W., Bica, K., Limbeck, A., 2019. FI-ICP-OES determination of Pb in drinking water after pre-concentration using magnetic nanoparticles coated with ionic liquid. Microchem. J. 146, 339–344 Hu, Z., Deibert, B.J., Li, J., 2014. Luminescent metal–organic frameworks for chemical sensing and explosive detection. Chem. Soc. Rev. 43, 5815–5840
Hua, Y., Kukkar, D., Brown, R.J., Kim, K.-H., 2022. Recent advances in the synthesis of and sensing applications for metal-organic framework-molecularly imprinted polymer (MOF-MIP) composites. Crit. Rev. Environ. Sci. Technol. 1–32
Huang, R.-F., Liu, H.-X., Gai, Q.-Q., Liu, G.-J., Wei, Z., 2015. A facile and sensitive electrochemiluminescence biosensor for Hg2+ analysis based on a dual-function oligonucleotide probe. Biosens. Bioelectron. 71, 194–199
Huangfu, M., Wang, M., Lin, C., Wang, J., Wu, P., 2021. Luminescent metal–organic frameworks as chemical sensors based on “mechanism–response”: a review. Dalton Trans. 50, 3429–3449
Isaeva, V., Tarasov, A., Starannikova, L., Yampol’skii, Y.P., Alent’ev, A.Y., Kustov, L., 2015. Microwave-assisted synthesis of mesoporous metal-organic framework NH2 MIL-101 (Al). Russ. Chem. Bull. 64, 2791–2795
Jayaramulu, K., Kanoo, P., George, S.J., Maji, T.K., 2010. Tunable emission from a porous metal–organic framework by employing an excited-state intramolecular proton transfer responsive ligand. Chem. Commun. 46, 7906–7908
Jeremias, F., Frohlich, D., Janiak, C., Henninger, S.K., 2014. Advancement of sorptionbased heat transformation by a metal coating of highly-stable, hydrophilic aluminium fumarate MOF. RSC Adv. 4, 24073–24082.
Johnson, M.L., Ackers, G.K., Holt, J.M., 1998. Energetics of Biological Macromolecules. Elsevier
Kalaj, M., Cohen, S.M., 2020. Postsynthetic modification: an enabling technology for the advancement of metal–organic frameworks. ACS Cent. Sci. 6, 1046–1057
Kalhor, S., Zarei, M., Zolfigol, M.A., Sepehrmansourie, H., Nematollahi, D., Alizadeh, S., Shi, H., Arjomandi, J., 2021. Anodic electrosynthesis of MIL-53 (Al)-N (CH2PO3H2) 2 as a mesoporous catalyst for synthesis of novel (N-methyl-pyrrol)-pyrazolo [3, 4-b] pyridines via a cooperative vinylogous anomeric based oxidation. Sci. Rep. 11, 1–20 Kamel, R.M., Shahat, A., Anwar, Z.M., El-Kady, H.A., Kilany, E.M., 2021. A novel sensitive and selective chemosensor for fluorescent detection of Zn 2+ in cosmetics creams based on a covalent post functionalized Al-MOF. N. J. Chem. 45, 8054–8063
Khan, N.A., Lee, J.S., Jeon, J., Jun, C.-H., Jhung, S.H., 2012. Phase-selective synthesis and phase-conversion of porous aluminum-benzenetricarboxylates with microwave irradiation. Microporous Mesoporous Mater. 152, 235–239
Khan, S., Guan, Q., Liu, Q., Qin, Z., Rasheed, B., Liang, X., Yang, X., 2021. Synthesis, modifications and applications of MILs metal-organic frameworks for environmental remediation: the cutting-edge review. Sci. Total Environ., 152279
Kim, H.S., Yu, K., Puthiaraj, P., Ahn, W.-S., 2020. CO2 cycloaddition to epichlorohydrin over an aluminum fumarate metal-organic framework synthesized by a sonochemical route. Microporous Mesoporous Mater. 306, 110432
Kim, J., Kim, W.Y., Ahn, W.-S., 2012. Amine-functionalized MIL-53 (Al) for CO2/N2 separation: effect of textural properties. Fuel 102, 574–579
Kim, S.-Y., Kang, J.H., Kim, S.-I., Bae, Y.-S., 2019. Extraordinarily large and stable methane delivery of MIL-53 (Al) under LNG-ANG conditions. Chem. Eng. J. 365, 242–248
Kreno, L.E., Leong, K., Farha, O.K., Allendorf, M., Van Duyne, R.P., Hupp, J.T., 2012. Metal–organic framework materials as chemical sensors. Chem. Rev. 112, 1105–1125
Krüger, M., Siegel, R., Dreischarf, A., Reinsch, H., Senker, J., Stock, N., 2015. [Al2 (OH) 2 (TCPB)]–an Al-MOF based on a tetratopic linker molecule. Microporous Mesoporous Mater. 216, 27–35
Lee, S., Kapustin, E.A., Yaghi, O.M., 2016. Coordinative alignment of molecules in chiral metal-organic frameworks. Science 353, 808–811
Lee, Y.-R., Kim, J., Ahn, W.-S., 2013. Synthesis of metal-organic frameworks: a mini review. Korean J. Chem. Eng. 30, 1667–1680
Lenzen, D., Bendix, P., Reinsch, H., Frohlich, D., Kummer, H., Mollers, M., Hügenell, P.P., Glaser, R., Henninger, S., Stock, N., 2018. Scalable green synthesis and full-scale test of the metal–organic framework CAU-10–H for use in adsorption-driven chillers. Adv. Mater. 30, 1705869
Lenzen, D., Zhao, J., Ernst, S.-J., Wahiduzzaman, M., Ken Inge, A., Frohlich, D., Xu, H., Bart, H.-J., Janiak, C., Henninger, S., 2019. A metal–organic framework for efficient water-based ultra-low-temperature-driven cooling. Nat. Commun. 10, 1–9
Leubner, S., Staglich, R., Franke, J., Jacobsen, J., Gosch, J., Siegel, R., Reinsch, H., Maurin, G., Senker, J., Yot, P.G., 2020. Solvent impact on the properties of benchmark metal–organic frameworks: acetonitrile-based synthesis of CAU-10, CeUiO-66, and Al-MIL-53. Chem. Eur. J. 26, 3877–3883
Li, C., Zhu, L., Yang, W., He, X., Zhao, S., Zhang, X., Tang, W., Wang, J., Yue, T., Li, Z., 2019. Amino-functionalized Al–MOF for fluorescent detection of tetracyclines in milk. J. Agric. Food Chem. 67, 1277–1283
Li, H.-Y., Zhao, S.-N., Zang, S.-Q., Li, J., 2020a. Functional metal–organic frameworks as effective sensors of gases and volatile compounds. Chem. Soc. Rev. 49, 6364–6401
Li, J., Hurlock, M.J., Goncharov, V.G., Li, X., Guo, X., Zhang, Q., 2021. Solvent-free and phase-selective synthesis of aluminum trimesate metal–organic frameworks. Inorg. Chem. 60, 4623–4632
Li, L., Chen, Y., Zhu, J.-J., 2017. Recent advances in electrochemiluminescence analysis. Anal. Chem. 89, 358–371
Li, P., Zhou, L.-J., Yang, N.-N., Sui, Q., Gong, T., Gao, E.-Q., 2018. Metal–organic frameworks with extended viologen units: metal-dependent photochromism, photomodulable fluorescence, and sensing properties. Cryst. Growth Des. 18, 7191–7198.
Li, S., Tan, L., Meng, X., 2020b. Nanoscale metal-organic frameworks: synthesis, biocompatibility, imaging applications, and thermal and dynamic therapy of tumors. Adv. Funct. Mater. 30, 1908924
Li, Z., Wu, Yn, Li, J., Zhang, Y., Zou, X., Li, F., 2015. The metal–organic framework MIL53 (Al) constructed from multiple metal sources: alumina, aluminum hydroxide, and boehmite. Chem. Eur. J. 21, 6913–6920
Lin, C., Zou, Z., Lei, Z., Wang, L., Song, Y., 2020. Fluorescent metal-organic frameworks MIL-101 (Al)-NH2 for rapid and sensitive detection of ellagic acid. Spectrochim. Acta Part A: Mol. Biomol. Spectrosc. 242, 118739
Liu, C., Yan, B., 2016. A novel photofunctional hybrid material of pyrene functionalized metal-organic framework with conformation change for fluorescence sensing of Cu2 + Sens. Actuators B: Chem. 235, 541–546
Liu, D., Lu, K., Poon, C., Lin, W., 2014. Metal–organic frameworks as sensory materials and imaging agents. Inorg. Chem. 53, 1916–1924
Liu, Y., Pan, M., Yang, Q.-Y., Fu, L., Li, K., Wei, S.-C., Su, C.-Y., 2012. Dual-emission from a single-phase Eu–Ag metal–organic framework: an alternative way to get whitelight phosphor. Chem. Mater. 24, 1954–1960
Liu, Y., Xie, X.-Y., Cheng, C., Shao, Z.-S., Wang, H.-S., 2019. Strategies to fabricate metal–organic framework (MOF)-based luminescent sensing platforms. J. Mater. Chem. C 7, 10743–10763
Lo, S.-H., Chien, C.-H., Lai, Y.-L., Yang, C.-C., Lee, J.J., Raja, D.S., Lin, C.-H., 2013. A mesoporous aluminium metal–organic framework with 3 nm open pores. J. Mater. Chem. A 1, 324–329
Lodeiro, C., Capelo, J.L., Oliveira, E., Lodeiro, J.F., 2019. New Toxic Emerging Contaminants: Beyond the Toxicological Effects. Springer, pp. 1–4.
Loiseau, T., Serre, C., Huguenard, C., Fink, G., Taulelle, F., Henry, M., Bataille, T., F´ erey, G., 2004. A rationale for the large breathing of the porous aluminum terephthalate (MIL-53) upon hydration. Chem. Eur. J. 10, 1373–1382
Loiseau, T., Mellot-Draznieks, C., Muguerra, H., Ferey, G., Haouas, M., Taulelle, F., 2005. Hydrothermal synthesis and crystal structure of a new three-dimensional aluminumorganic framework MIL-69 with 2, 6-naphthalenedicarboxylate (ndc), Al (OH)(ndc)⋅ H2O. Comptes Rendus Chim. 8, 765–772
Loiseau, T., Lecroq, L., Volkringer, C., Marrot, J., Ferey, G., Haouas, M., Taulelle, F., Bourrelly, S., Llewellyn, P.L., Latroche, M., 2006. MIL-96, a porous aluminum trimesate 3D structure constructed from a hexagonal network of 18-membered rings and μ 3-oxo-centered trinuclear units. J. Am. Chem. Soc. 128, 10223–10230
Loiseau, T., Volkringer, C., Haouas, M., Taulelle, F., Ferey, G., 2015. Crystal chemistry of aluminium carboxylates: From molecular species towards porous infinite threedimensional networks. Comptes Rendus Chim. 18, 1350–1369
Lu, L., Guo, L., Li, J., Kang, T., Cheng, S., 2016. Electrochemiluminescent detection of Pb2+ by graphene/gold nanoparticles and CdSe quantum dots. Appl. Surf. Sci. 388, 431–436
Lu, M., Deng, Y., Luo, Y., Lv, J., Li, T., Xu, J., Chen, S.-W., Wang, J., 2018. Graphene aerogel–metal–organic framework-based electrochemical method for simultaneous detection of multiple heavy-metal ions. Anal. Chem. 91, 888–895
Lu, Y., Yan, B., Liu, J.-L., 2014. Nanoscale metal–organic frameworks as highly sensitive luminescent sensors for Fe 2+ in aqueous solution and living cells. Chem. Commun. 50, 9969–9972.
Lu, Y., Liu, C., Mei, C., Sun, J., Lee, J., Wu, Q., Hubbe, M.A., Li, M.-C., 2022. Recent advances in metal organic framework and cellulose nanomaterial composites. Coord. Chem. Rev. 461, 214496
Luo, J., Liu, B.-S., Zhang, X.-R., Liu, R.-T., 2019. A Eu3+ post-functionalized metalorganic framework as fluorescent probe for highly selective sensing of Cu2+ in aqueous media. J. Mol. Struct. 1177, 444–448
Lustig, W.P., Mukherjee, S., Rudd, N.D., Desai, A.V., Li, J., Ghosh, S.K., 2017. Metal–organic frameworks: functional luminescent and photonic materials for sensing applications. Chem. Soc. Rev. 46, 3242–3285
Lv, R., Chen, Z., Fu, X., Yang, B., Li, H., Su, J., Gu, W., Liu, X., 2018. A highly selective and fast-response fluorescent probe based on Cd-MOF for the visual detection of Al3 + ion and quantitative detection of Fe3+ ion. J. Solid State Chem. 259, 67–72
Ma, X., Zhang, X., Han, L., Hao, Z., Yong, S., Multi-response, A., 2021. Aluminum metalorganic frameworks for fluorescence sensing of Fe3+, Sr2+, SiO3 2 and toluene. Methods Appl. Fluoresc. 9, 025007
Mahata, S., Bhattacharya, A., Kumar, J.P., Mandal, B.B., Manivannan, V., 2020. Nakedeye detection of Pd2+ ion using a highly selective fluorescent heterocyclic probe by “turn-off” response and in-vitro live cell imaging. J. Photochem. Photobiol. A: Chem. 394, 112441
Majhi, S.M., Ali, A., Rai, P., Greish, Y., Alzamly, A., Surya, S.G., Qamhieh, N., Mahmoud, S.T., 2022. Metal-organic frameworks for advanced transducers based gas sensors: review and perspectives. Nanoscale Adv.
Mandal, S., Natarajan, S., Mani, P., Pankajakshan, A., 2021. Post-synthetic modification of metal–organic frameworks toward applications. Adv. Funct. Mater. 31, 2006291.
Martinez Joaristi, A., Juan-Alcaniz, J., Serra-Crespo, P., Kapteijn, F., Gascon, J., 2012. Electrochemical synthesis of some archetypical Zn2+, Cu2+, and Al3+ metal organic frameworks. Cryst. Growth Des. 12, 3489–3498
McLeod, C.L., Shaulis, B.J., 2018. Rare earth elements in planetary crusts: insights from chemically evolved igneous suites on Earth and the Moon. Minerals 8, 455
Moreau, F., Marrot, J., Banse, F., Serre, C., Tissot, A., 2020. Sequential installation of Fe (ii) complexes in MOFs: towards the design of solvatochromic porous solids.
J. Mater. Chem. C. 8, 16826–16833
Nandi, S., Mondal, A., Reinsch, H., Biswas, S., 2019. An ultra-robust luminescent CAU-10 MOF acting as a fluorescent “turn-off” sensor for Cr2O72 in aqueous medium. Inorg. Chim. Acta 497, 119078
Nandi, S., Reinsch, H., Biswas, S., 2020. A vinyl functionalized mixed linker CAU-10 metal-organic framework acting as a fluorescent sensor for the selective detection of H2S and palladium (II). Microporous Mesoporous Mater. 293, 109790.
Niekiel, F., Ackermann, M., Guerrier, P., Rothkirch, A., Stock, N., 2013. Aluminum-1, 4cyclohexanedicarboxylates: High-throughput and temperature-dependent in situ EDXRD studies. Inorg. Chem. 52, 8699–8705
Niekiel, F., Lannoeye, J., Reinsch, H., Munn, A.S., Heerwig, A., Zizak, I., Kaskel, S., Walton, R.I., De Vos, D., Llewellyn, P., 2014. Conformation-controlled sorption properties and breathing of the aliphatic Al-MOF [Al (OH)(CDC)]. Inorg. Chem. 53, 4610–4620
Paixao, T.R.L.C., Reddy, S.M., 2017. Materials for Chemical Sensing. Springer
Paolesse, R., Nardis, S., Monti, D., Stefanelli, M., Di Natale, C., 2017. Porphyrinoids for chemical sensor applications. Chem. Rev. 117, 2517–2583
Parmar, B., Rachuri, Y., Bisht, K.K., Suresh, E., 2017. Mixed-ligand LMOF fluorosensors for detection of Cr (VI) oxyanions and Fe3+/Pd2+ cations in aqueous media. Inorg. Chem. 56, 10939–10949
Pei, L., Su, J., Yang, H., Wu, Y., Du, Y., Zhu, Y., 2022. A novel covalent-organic framework for highly sensitive detection of Cd2+, Pb2+, Cu2+ and Hg2.
Microporous Mesoporous Mater. 333, 111742
Pellow, R., Vala, M., 1989. The external heavy atom effEct: Theory of spin–orbit coupling of alkali and noble metals in rare gas matrices. J. Chem. Phys. 90, 5612–5621
Pereira, L.C., de Souza, A.O., Bernardes, M.F.F., Pazin, M., Tasso, M.J., Pereira, P.H., Dorta, D.J., 2015. A perspective on the potential risks of emerging contaminants to human and environmental health. Environ. Sci. Pollut. Res. 22, 13800–13823
Permyakova, A., Skrylnyk, O., Courbon, E., Affram, M., Wang, S., Lee, U.H., Valekar, A. H., Nouar, F., Mouchaham, G., Devic, T., 2017. Synthesis optimization, shaping, and heat reallocation evaluation of the hydrophilic metal–organic framework MIL-160 (Al). ChemSusChem 10, 1419–1426.
Qin, Y.-y, Wang, Q.-y, Ge, J.-L., Wu, F., 2021. Microwave ultrasound-assisted synthesis of NH2-MIL-53 (Al) for fluorescence detection of organosulfur compounds in model fuel. Inorg. Chem. Commun. 132, 108828
A. Rahmani, A. Shabanloo, S. Zabihollahi, M. Salari, M. Leili, M. Khazaei, S. Alizadeh, D. Nematollahi, Facile Fabrication of Amino-Functionalized MIL-68 (Al) Metal-organic Framework for Effective Adsorption of Arsenate (As (V)), (2022).
Raja, D.S., Chang, I.-H., Jiang, Y.-C., Chen, H.-T., Lin, C.-H., 2015. Enhanced gas sorption properties of a new sulfone functionalized aluminum metal-organic framework: Synthesis, characterization, and DFT studies. Microporous Mesoporous Mater. 216, 20–26
Raju, C.V., Kumar, S.S., 2021. Co-reactant-free self-enhanced solid-state electrochemiluminescence platform based on polyluminol-gold nanocomposite for signal-on detection of mercury ion. Sci. Rep. 11, 1–11
Ramasamy, K., Thambusamy, S., 2017. Dual emission and pH based naphthalimide derivative fluorescent sensor for the detection of Bi3+ Sens. Actuators B: Chem. 247, 632–640
Ramírez-Malule, H., Quinones-Murillo, D.H., Manotas-Duque, D., 2020. Emerging contaminants as global environmental hazards. A bibliometric analysis. Emerg. Contam. 6, 179–193
Raptopoulou, C.P., 2021. Metal-organic frameworks: Synthetic methods and potential applications. Materials 14, 310
Reimer, N., Reinsch, H., Inge, A.K., Stock, N., 2015. New Al-MOFs based on sulfonyldibenzoate ions: a rare example of intralayer porosity. Inorg. Chem. 54, 492–501.
Reinsch, H., Feyand, M., Ahnfeldt, T., Stock, N., 2012a. CAU-3: A new family of porous MOFs with a novel Al-based brick:[Al 2 (OCH 3) 4 (O 2 CX-CO 2)](X= aryl). Dalton Trans. 41, 4164–4171
Reinsch, H., Marszałek, B., Wack, J., Senker, J., Gil, B., Stock, N., 2012b. A new Al-MOF based on a unique column-shaped inorganic building unit exhibiting strongly hydrophilic sorption behaviour. Chem. Commun. 48, 9486–9488
Reinsch, H., Kruger, M., Marrot, J., Stock, N., 2013a. First keto-functionalized microporous al-based metal–organic framework:[Al (OH)(O2C-C6H4-CO-C6H4CO2)]. Inorg. Chem. 52, 1854–1859
Reinsch, H., van der Veen, M.A., Gil, B., Marszalek, B., Verbiest, T., De Vos, D., Stock, N., 2013b. Structures, sorption characteristics, and nonlinear optical properties of a new series of highly stable aluminum MOFs. Chem. Mater. 25, 17–26
Reinsch, H., De Vos, D., Stock, N., 2013c. Structure and Properties of [Al4 (OH) 8 (oC6H4 (CO2) 2) 2] H2O, a layered aluminum phthalate. Z. Anorg. Allg. Chem. 639, 2785–2789
Ren, M., Wang, H., Liu, Y., Ma, Q., Jia, W., Liu, M., Wang, H., Lu, Y., 2020. Fluorescent determination of mercury (II) and glutathione using amino-MIL-53 (Al) nanosheets. Anal. Lett. 53, 2700–2714
Rubio-Martinez, M., Avci-Camur, C., Thornton, A.W., Imaz, I., Maspoch, D., Hill, M.R., 2017. New synthetic routes towards MOF production at scale. Chem. Soc. Rev. 46, 3453–3480
Safaei, M., Foroughi, M.M., Ebrahimpoor, N., Jahani, S., Omidi, A., Khatami, M., 2019. A review on metal-organic frameworks: synthesis and applications. TrAC Trends Anal. Chem. 118, 401–425
Samokhvalov, A., 2018. Aluminum metal–organic frameworks for sorption in solution: a review. Coord. Chem. Rev. 374, 236–253
Schilling, L.H., Reinsch, H., Stock, N., 2016. Synthesis, structure, and selected properties of aluminum-, gallium-, and indium-based metal–organic frameworks, the chemistry of metal–organic frameworks: synthesis. Charact., Appl. 1, 105–135
Schlusener, C., Xhinovci, M., Ernst, S.-J., Schmitz, A., Tannert, N., Janiak, C., 2019. Solid-solution mixed-linker synthesis of isoreticular Al-based MOFs for an easy hydrophilicity tuning in water-sorption heat transformations. Chem. Mater. 31, 4051–4062
Schlüsener, C., Jordan, D.N., Xhinovci, M., Ntep, T.J.M.M., Schmitz, A., Giesen, B., Janiak, C., 2020. Probing the limits of linker substitution in aluminum MOFs through water vapor sorption studies: mixed-MOFs instead of mixed-linker CAU-23 and MIL-160 materials. Dalton Trans. 49, 7373–7383
Schneemann, A., Bon, V., Schwedler, I., Senkovska, I., Kaskel, S., Fischer, R.A., 2014. Flexible metal–organic frameworks. Chem. Soc. Rev. 43, 6062–6096
Schroeder, V., Savagatrup, S., He, M., Lin, S., Swager, T.M., 2018. Carbon nanotube chemical sensors. Chem. Rev. 119, 599–663
Sen, B., Kumar Patra, S., Rabha, M., Kumar Sheet, S., Aguan, K., Samanta, D., Khatua, S., 2021. Luminescence detection of Ag+ and phosphate ions by a ruthenium (II) complex-based multianalyte probe: a combined spectroscopic, crystallographic, and theoretical approach. Eur. J. Inorg. Chem. 2021, 3549–3560
Senkovska, I., Hoffmann, F., Froba, M., Getzschmann, J., Bohlmann, W., Kaskel, S., 2009. New highly porous aluminium based metal-organic frameworks: Al (OH)(ndc)(ndc= 2, 6-naphthalene dicarboxylate) and Al (OH)(bpdc)(bpdc= 4, 4′ -biphenyl dicarboxylate). Microporous Mesoporous Mater. 122, 93–98
Seoane, B., Dikhtiarenko, A., Mayoral, A., Tellez, C., Coronas, J., Kapteijn, F., Gascon, J., 2015. Metal organic framework synthesis in the presence of surfactants: towards hierarchical MOFs? CrystEngComm 17, 1693–1700
Serra-Crespo, P., Ramos-Fernandez, E.V., Gascon, J., Kapteijn, F., 2011. Synthesis and characterization of an amino functionalized MIL-101 (Al): separation and catalytic properties. Chem. Mater. 23, 2565–2572
Shao, J.-J., Ni, J.-L., Liang, Y., Li, G.-J., Chen, L.-Z., Wang, F.-M., 2022. Luminescent MOFs for selective sensing of Ag+ and other ions (Fe (iii) and Cr (vi)) in aqueous solution. CrystEngComm 24, 2479–2484
Shi, J., Han, R., Lu, S., Liu, Q., metal-OH, A., 2021a. group modification strategy to prepare highly-hydrophobic MIL-53-Al for efficient acetone capture under humid conditions. J. Environ. Sci. 107, 111–123
Shi, L., Li, N., Wang, D., Fan, M., Zhang, S., Gong, Z., 2021b. Environmental pollution analysis based on the luminescent metal organic frameworks: a review. TrAC Trends Anal. Chem. 134, 116131
Sikka, R., Kumar, P., Lee, J., Sonne, C., 2022. Aqueous-phase biofunctionalized NH2MIL-53 (Al) MOF for biosensing applications. J. Porous Mater. 1–8
Stavitski, E., Goesten, M., Juan-Alcaniz, J., Martinez-Joaristi, A., Serra-Crespo, P., Petukhov, A.V., Gascon, J., Kapteijn, F., 2011. Kinetic control of metal–organic framework crystallization investigated by time-resolved in situ X-Ray scattering. Angew. Chem. 123, 9798–9802
Steenhaut, T., Filinchuk, Y., Hermans, S., 2021. Aluminium-based MIL-100 (Al) and MIL101 (Al) metal–organic frameworks, derivative materials and composites: synthesis, structure, properties and applications. J. Mater. Chem. A 9, 21483–21509
Stock, N., 2014. Synthesis and structures of aluminum-based metal-organic frameworks. Metal. Org. Framew. Mater. 1–16
Stock, N., Biswas, S., 2012. Synthesis of metal-organic frameworks (MOFs): routes to various MOF topologies, morphologies, and composites. Chem. Rev. 112, 933–969 Sud, D., Kaur, G., 2021. A comprehensive review on synthetic approaches for metalorganic frameworks: From traditional solvothermal to greener protocols. Polyhedron 193, 114897
Sun, L., Yin, M., Li, Z., Tang, S., 2022. Facile microwave-assisted solvothermal synthesis of rod-like aluminum terephthalate [MIL-53 (Al)] for CO2 adsorption. J. Ind. Eng. Chem.
Sun, Y., Zhou, H.-C., 2015. Recent progress in the synthesis of metal–organic frameworks. Sci. Technol. Adv. Mater.
Taheri, A., Babakhani, E.G., Towfighi, J., 2018. Study of synthesis parameters of MIL-53 (Al) using experimental design methodology for CO2/CH4 separation. Adsorpt. Sci. Technol. 36, 247–269
Tannert, N., Jansen, C., Nießing, S., Janiak, C., 2019. Robust synthesis routes and porosity of the Al-based metal–organic frameworks Al-fumarate, CAU-10-H and MIL160. Dalton Trans. 48, 2967–2976
Tehrani, M.S., Zare-Dorabei, R., 2016a. Competitive removal of hazardous dyes from aqueous solution by MIL-68 (Al): derivative spectrophotometric method and response surface methodology approach. Spectrochim. Acta Part A Mol. Biomol. Spectrosc. 160, 8–18
Tehrani, M.S., Zare-Dorabei, R., 2016b. Highly efficient simultaneous ultrasonic-assisted adsorption of methylene blue and rhodamine B onto metal organic framework MIL68 (Al): central composite design optimization. RSC Adv. 6, 27416–27425
Thomas-Hillman, I., Stevens, L.A., Lange, M., Mollmer, J., Lewis, W., Dodds, C., Kingman, S.W., Laybourn, A., 2019. Developing a sustainable route to environmentally relevant metal–organic frameworks: ultra-rapid synthesis of MFM300 (Al) using microwave heating. Green. Chem. 21, 5039–5045
Tricoli, A., Righettoni, M., Teleki, A., 2010. Semiconductor gas sensors: dry synthesis and application. Angew. Chem. Int. Ed. 49, 7632–7659
Vinu, M., Lin, W.-C., Senthil Raja, D., Han, J.-L., Lin, C.-H., 2017. Microwave-assisted synthesis of nanoporous aluminum-based coordination polymers as catalysts for selective sulfoxidation reaction. Polymers 9, 498.
Voica, C., Dehelean, A., Pamula, A., 2009. Method validation for determination of heavy metals in wine and slightly alcoholic beverages by ICP-MS. In: Journal of Physics: Conference Series. IOP Publishing, 012036
Volkringer, C., Popov, D., Loiseau, T., Guillou, N., Ferey, G., Haouas, M., Taulelle, F., Mellot-Draznieks, C., Burghammer, M., Riekel, C., 2007. A microdiffraction set-up for nanoporous metal–organic-framework-type solids. Nat. Mater. 6, 760–764
Volkringer, C., Loiseau, T., Guillou, N., F´ erey, G., Haouas, M., Taulelle, F., Audebrand, N., Margiolaki, I., Popov, D., Burghammer, M., 2009a. Structural transitions and flexibility during dehydration rehydration process in the MOF-type aluminum pyromellitate Al2 (OH) 2 [C10O8H2](MIL-118). Cryst. Growth Des. 9, 2927–2936
Volkringer, C., Loiseau, T., Haouas, M., Taulelle, F., Popov, D., Burghammer, M., Riekel, C., Zlotea, C., Cuevas, F., Latroche, M., 2009a. Occurrence of uncommon infinite chains consisting of edge-sharing octahedra in a porous metal organic framework-type aluminum pyromellitate Al4 (OH) 8 [C10O8H2](MIL-120): synthesis, structure, and gas sorption properties. Chem. Mater. 21, 5783–5791
Volkringer, C., Loiseau, T., Guillou, N., F´ erey, G., Elkaïm, E., 2009b. Syntheses and structures of the MOF-type series of metal 1, 4, 5, 8,-naphthalenetetracarboxylates M2 (OH) 2 [C14O8H4](Al, Ga, In) with infinite trans-connected M–OH–M chains (MIL-122). Solid State Sci. 11, 1507–1512
Volkringer, C., Loiseau, T., Guillou, N., Ferey, G., Haouas, M., Taulelle, F., Elkaim, E., Stock, N., 2010a. High-throughput aided synthesis of the porous metal organic framework-type aluminum pyromellitate, MIL-121, with extra carboxylic acid functionalization. Inorg. Chem. 49, 9852–9862
Volkringer, C., Loiseau, T., Devic, T., Ferey, G., Popov, D., Burghammer, M., Riekel, C., 2010b. A layered coordination polymer based on an azodibenzoate linker connected to aluminium (MIL-129). CrystEngComm 12, 3225–3228
Volkringer, C., Loiseau, T., Guillou, N., Ferey, G., Popov, D., Burghammer, M., Riekel, C., 2013. Synthesis and structural characterization of metal–organic frameworks with the mellitate linker M2 (OH) 2 [C12O12H2] 2H2O (M= Al, Ga, In) MIL-116. Solid State Sci. 26, 38–44
Wan, J., Shen, Y., Xu, L., Xu, R., Zhang, J., Sun, H., Zhang, C., Yin, C., Wang, X., 2021. Ferrocene-functionalized Ni (II)-based metal-organic framework as electrochemical sensing interface for ratiometric analysis of Cu2+, Pb2+ and Cd2. J. Electroanal. Chem. 895, 115374
Wang, B., Yang, Q., Guo, C., Sun, Y., Xie, L.-H., Li, J.-R., 2017. Stable Zr (IV)-based metal–organic frameworks with predesigned functionalized ligands for highly selective detection of Fe (III) ions in water. ACS Appl. Mater. Interfaces 9, 10286–10295.
Wang, H., Chen, Q., Tan, Z., Yin, X., Wang, L., 2012. Electrochemiluminescence of CdTe quantum dots capped with glutathione and thioglycolic acid and its sensing of Pb2. Electrochim. Acta 72, 28–31
Wang, L., Luo, D., Qin, D., Shan, D., Lu, X., 2015b. Cathodic electrochemiluminescence of a CdSe/ZnS QDs-modified glassy carbon electrode and its application in sensing of Pb 2+ Anal. Methods 7, 1395–1400
Wang, L., Zheng, X.-Y., Zhang, X., Zhu, Z.-J., 2021. A quinoline-based fluorescent chemosensor for palladium ion (Pd2+)-selective detection in aqueous solution. Spectrochim. Acta Part A: Mol. Biomol. Spectrosc. 249, 119283
Wang, N., Zhao, W., Shen, Z., Sun, S., Dai, H., Ma, H., Lin, M., 2020b. Sensitive and selective detection of Pb (II) and Cu (II) using a metal-organic framework/ polypyrrole nanocomposite functionalized electrode. Sens. Actuators B: Chem. 304, 127286
Wang, Z., Cohen, S.M., 2009. Postsynthetic modification of metal–organic frameworks. Chem. Soc. Rev. 38, 1315–1329
Wang, Z., Babucci, M., Zhang, Y., Wen, Y., Peng, L., Yang, B., Gates, B.C., Yang, D., 2020a. Dialing in catalytic sites on metal organic framework nodes: MIL-53 (Al) and MIL-68 (Al) probed with methanol dehydration catalysis. ACS Appl. Mater. Interfaces 12, 53537–53546
Wang, Z.W., Chen, M., Liu, C.S., Wang, X., Zhao, H., Du, M., Versatile, A., 2015a. AlIIIbased metal–organic framework with high physicochemical stability. Chem. Eur. J. 21, 17215–17219
Wilkinson, J., Hooda, P.S., Barker, J., Barton, S., Swinden, J., 2017. Occurrence, fate and transformation of emerging contaminants in water: an overarching review of the field. Environ. Pollut. 231, 954–970
Wu, R., Ai, J., Ga, L., 2022. Synthesis of fluorescent copper nanomaterials and detection of Bi3+ Front. Chem. 10
Wu, T., Prasetya, N., Li, K., 2020. Recent advances in aluminium-based metal-organic frameworks (MOF) and its membrane applications. J. Membr. Sci. 615, 118493
Xiao-Ping, W., Feng, Y., Fan, D., Jian-Ping, L., 2014. An electrochemiluminescence biosensor for determination of Pb2+ based on G-quadruplex of aptamer probe. Chin. J. Anal. Chem. 42, 942–947
Xie, D.-h, Ge, X., Qin, W.-x, Zhang, Y.-x, 2021. NH2-MIL-53 (Al) for simultaneous removal and detection of fluoride anions. Chin. J. Chem. Phys. 34, 227–237
Xu, L., Gan, S., Zhong, L., Sun, Z., Tang, Y., Han, T., Lin, K., Liao, C., He, D., Ma, Y., 2022. Conductive metal organic framework for ion-selective membrane-free solid-contact potentiometric Cu2+ sensing. J. Electroanal. Chem. 904, 115923
Xu, X.-Y., Yan, B., 2016. Fabrication and application of a ratiometric and colorimetric fluorescent probe for Hg 2+ based on dual-emissive metal–organic framework hybrids with carbon dots and Eu 3. J. Mater. Chem. C 4, 1543–1549
Yan, B., 2017. Lanthanide-functionalized metal–organic framework hybrid systems to create multiple luminescent centers for chemical sensing. Acc. Chem. Res. 50, 2789–2798
Yang, C.-X., Ren, H.-B., Yan, X.-P., 2013. Fluorescent metal–organic framework MIL-53 (Al) for highly selective and sensitive detection of Fe3+ in aqueous solution. Anal. Chem. 85, 7441–7446
Yang, G.L., Jiang, X.L., Xu, H., Zhao, B., 2021. Applications of MOFs as luminescent sensors for environmental pollutants. Small 17, 2005327
Yang, J., Wang, J., Deng, S., Li, J., 2016. Improved synthesis of trigone trimer cluster metal organic framework MIL-100Al by a later entry of methyl groups. Chem. Commun. 52, 725–728.
Yang, Q., Vaesen, S., Vishnuvarthan, M., Ragon, F., Serre, C., Vimont, A., Daturi, M., De Weireld, G., Maurin, G., 2012b. Probing the adsorption performance of the hybrid porous MIL-68 (Al): a synergic combination of experimental and modelling tools. J. Mater. Chem. 22, 10210–10220
Yang, S., Sun, J., Ramirez-Cuesta, A.J., Callear, S.K., David, W.I., Anderson, D.P., Newby, R., Blake, A.J., Parker, J.E., Tang, C.C., 2012a. Selectivity and direct visualization of carbon dioxide and sulfur dioxide in a decorated porous host. Nat. Chem. 4, 887–894
Yang, X., Wang, Y., Liu, R., Zhang, Y., Tang, J., Yang, E.-B., Zhang, D., Zhao, Y., Ye, Y., 2019. A novel ICT-based two photon and NIR fluorescent probe for labile Fe2+ detection and cell imaging in living cells. Sens. Actuators B Chem. 288, 217–224
Yin, H.-Q., Yang, J.-C., Yin, X.-B., 2017. Ratiometric fluorescence sensing and real-time detection of water in organic solvents with one-pot synthesis of Ru@ MIL-101 (Al)–NH2. Anal. Chem. 89, 13434–13440
Yin, Z., Wan, S., Yang, J., Kurmoo, M., Zeng, M.-H., 2019. Recent advances in postsynthetic modification of metal–organic frameworks: New types and tandem reactions. Coord. Chem. Rev. 378, 500–512
Younis, S.A., Bhardwaj, N., Bhardwaj, S.K., Kim, K.-H., Deep, A., 2021. Rare earth metal–organic frameworks (RE-MOFs): Synthesis, properties, and biomedical applications. Coord. Chem. Rev. 429, 213620
Yuan, S., Feng, L., Wang, K., Pang, J., Bosch, M., Lollar, C., Sun, Y., Qin, J., Yang, X., Zhang, P., 2018. Stable metal–organic frameworks: design, synthesis, and applications. Adv. Mater. 30, 1704303
Zhang, C., Pan, G., He, Y., 2022b. Conjugated microporous organic polymer as fluorescent chemosensor for detection of Fe3+ and Fe2+ ions with high selectivity and sensitivity. Talanta 236, 122872
Zhang, E., Ju, P., Li, Q., Hou, X., Yang, H., Yang, X., Zou, Y., Zhang, Y., 2018a. A novel rhodamine 6G-based fluorescent and colorimetric probe for Bi3+: synthesis, selectivity, sensitivity and potential applications. Sens. Actuators B: Chem. 260, 204–212
Zhang, H., Hu, X., Li, T., Zhang, Y., Xu, H., Sun, Y., Gu, X., Gu, C., Luo, J., Gao, B., 2022a. MIL series of metal organic frameworks (MOFs) as novel adsorbents for heavy metals in water: a review. J. Hazard. Mater., 128271
Zhang, L., Wang, J., Ren, X., Zhang, W., Zhang, T., Liu, X., Du, T., Li, T., Wang, J., 2018b. Internally extended growth of core–shell NH 2-MIL-101 (Al)@ ZIF-8 nanoflowers for the simultaneous detection and removal of Cu (ii). J. Mater. Chem. A 6, 21029–21038
Zhang, L., Wang, J., Du, T., Zhang, W., Zhu, W., Yang, C., Yue, T., Sun, J., Li, T., Wang, J., 2019a. NH2-MIL-53 (Al) metal–organic framework as the smart platform for simultaneous high-performance detection and removal of Hg2+ Inorg. Chem. 58, 12573–12581
Zhang, L., Liu, H., Shi, W., Cheng, P., 2019b. Synthesis strategies and potential applications of metal-organic frameworks for electrode materials for rechargeable lithium ion batteries. Coord. Chem. Rev. 388, 293–309
Zhang, M., Ge, L., Ge, S., Yan, M., Yu, J., Huang, J., Liu, S., 2013. Three-dimensional paper-based electrochemiluminescence device for simultaneous detection of Pb2+ and Hg2+ based on potential-control technique. Biosens. Bioelectron. 41, 544–550
Zhang, Y., Yan, B., 2019. A ratiometric fluorescent sensor with dual response of Fe3+/ Cu2+ based on europium post-modified sulfone-metal-organic frameworks and its logical application. Talanta 197, 291–298
Zhao, Z.-P., Jiang, Y.-F., Chen, Y., Li, H.-R., Zheng, Y., Zeng, C.-H., Zhong, S., Guo, P., Zhao, Y.-L., 2018. Highly luminescent lanthanide complex as bifunctional sensor for Et2O and Fe2. J. Lumin. 204, 560–567
Zhou, H.-C., Long, J.R., Yaghi, O.M., 2012. Introduction to Metal–organic Frameworks. ACS Publications, pp. 673–674 (in)
Zhou, Y., Chen, H.-H., Yan, B., 2014. An Eu 3+ post-functionalized nanosized metal–organic framework for cation exchange-based Fe 3+-sensing in an aqueous environment. J. Mater. Chem. A 2, 13691–13697
Zhu, Z., He, X., Wang, W.-N., 2020. Unraveling the origin of the “Turn-On” effect of AlMIL-53-NO 2 during H 2 S detection. CrystEngComm 22, 195–204