NANOCELLULOSE MATERIALS
Fabrication and Industrial Applications
Edited by
RAMESH
ORAON
Department of Nanoscience and Technology, Central University of Jharkhand, Brambe, Ranchi, Jharkhand, India
DEEPAK RAWTANI
School of Pharmacy, National Forensic Sciences University (Ministry of Home Affairs, GOI), Gandhinagar, Gujarat, India
PARDEEP SINGH
Department of Environmental Studies, PGDAV College, University of Delhi, New Delhi, India
CHAUDHERY MUSTANSAR HUSSAIN
Department of Chemistry and Environmental Science, New Jersey Institute of Technology, Newark, New Jersey, USA
Elsevier
Radarweg 29, PO Box 211, 1000 AE Amsterdam, Netherlands
The Boulevard, Langford Lane, Kidlington, Oxford OX5 1GB, United Kingdom 50 Hampshire Street, 5th Floor, Cambridge, MA 02139, United States
Copyright © 2022 Elsevier Inc. All rights reserved.
No part of this publication may be reproduced or transmitted in any form or by any means, electronic or mechanical, including photocopying, recording, or any information storage and retrieval system, without permission in writing from the publisher. Details on how to seek permission, further information about the Publisher’s permissions policies and our arrangements with organizations such as the Copyright Clearance Center and the Copyright Licensing Agency, can be found at our website: www.elsevier.com/permissions
This book and the individual contributions contained in it are protected under copyright by the Publisher (other than as may be noted herein).
Notices
Knowledge and best practice in this field are constantly changing. As new research and experience broaden our understanding, changes in research methods, professional practices, or medical treatment may become necessary.
Practitioners and researchers must always rely on their own experience and knowledge in evaluating and using any information, methods, compounds, or experiments described herein. In using such information or methods they should be mindful of their own safety and the safety of others, including parties for whom they have a professional responsibility.
To the fullest extent of the law, neither the Publisher nor the authors, contributors, or editors, assume any liability for any injury and/or damage to persons or property as a matter of products liability, negligence or otherwise, or from any use or operation of any methods, products, instructions, or ideas contained in the material herein.
British Library Cataloguing-in-Publication Data
A catalogue record for this book is available from the British Library
Library of Congress Cataloging-in-Publication Data
A catalog record for this book is available from the Library of Congress
ISBN: 978-0-12-823963-6
For Information on all Elsevier publications visit our website at https://www.elsevier.com/books-and-journals
Publisher: Matthew Deans
Acquisitions Editor: Simon Holt
Editorial Project Manager: Gabriela D. Capille
Production Project Manager: Surya Narayanan Jayachandran
Cover Designer: Miles Hitchen
Typeset by Aptara, New Delhi, India
4.
1. Bacterial cellulose nanofibers for
Emel Tamahkar, Aykut Arif Topçu, Işık Perçin, Sevgi Aslıyüce, Adil Denizli
1.1
1.4
2.
Mani Pujitha Illa,
Adepu, Mudrika Khandelwal
3.1
Nadia Obrownick Okamoto-Schalch, Natalia Cristina da Silva, Rafael Belasque Canedo da Silva, Milena Martelli Tosi
4.1
4.3
5. Recent developments of bacterial nanocellulose porous scaffolds in biomedical applications
Swaminathan Jiji, Kannan Maharajan, Krishna Kadirvelu
5.1
5.2 Properties
5.3 Importance of BNC
5.4
5.5
6. Characteristic features and functions of nanocellulose for its feasible application in textile industry
P. R Sreeraj, Santosh Kr. Mishra, Purushottam Kumar Singh
6.1 Introduction
6.2
6.3
6.4
6.5 General techniques
6.6 Pretreatment of nanocellulose
6.7 Characterization and thermal analysis of nanocellulose particles
6.8 Effects of nanocellulose on the properties of the textiles
6.9 Applications, advantages, and limitations of nanocellulose
6.10 Current trends and future scopes
6.11
7. Nanocellulose in plastic industry 123 Sapna Jain, Bhawna Yadav Lamba, Sanjeev Kumar
7.1 Introduction
7.2
7.3 Bioplastics
7.4 Cellulose
7.5 Nanocellulosic composites: potential
7.6
8.
Archana Singh, Deepak Rawtani, Shruti Jha
9.
Garvita Parikh, Bansari Parikh, Aarohi Natu, Deepak Rawtani
9.1
9.3
9.6
9.7
9.8
9.9
9.10
11.
Birendra Bharti, Vibhanshu Kumar, Himanshu Kumar
Benjamin Raj, Mamata Mohapatra, Arun. K. Padhy, Suddhasatwa Basu
12.
Athanasia Amanda Septevani, Dian Burhani, Yulianti Sampora
12.1
12.3
12.4
12.5
12.6
13.
Mansi Chugh, Tulsi Chandak, Shruti Jha, Deepak Rawtani
13.1
13.7
13.8
13.9
14.
Gurudatta Singh, Syed Saquib, Ankita Gupta, Swati 14.1
14.6
14.7
14.8
14.9
14.10
15.
Anamika
15.1
15.2
Shalini Tirkey, Shashikant Shivaji Vhatkar, Ramesh Oraon
15.3 Unmanned
15.4
15.5
15.6
15.7
Contributors
Shivakalyani Adepu
Cellulose and composites group, Department of Materials Science and Metallurgical Engineering, IIT Hyderabad, Telangana, India
Sevgi Aslıyüce
Department of Chemistry, Hacettepe University, Beytepe, Ankara, Turkey
Suddhasatwa Basu
CSIR-Institute of Minerals and Materials Technology, Bhubaneswar, Odisha, India
Birendra Bharti
Department of Water Engineering and Management, Central University of Jharkhand, Jharkhand, India
Dian Burhani
Research Center for Biomaterial – Indonesian Institute of Sciences (LIPI), Cibinong, Bogor, Indonesia
Tulsi Chandak
School of Engineering and Technology, National Forensic Sciences University (Ministry of Home affairs, GOI), Gandhinagar, Gujarat, India
Mansi Chugh
School of Engineering and Technology, National Forensic Sciences University (Ministry of Home affairs, GOI), Gandhinagar, Gujarat, India
Rafael Belasque Canedo da Silva
Departamento de Engenharia de Alimentos, Faculdade de Zootecnia e Engenharia de Alimentos, Universidade de São Paulo, Rua Duque de Caxias Norte 225, Pirassununga, SP, Brazil; Postgraduate Programme in Materials Science and Engineering, University of São Paulo, USP/ FZEA, Av. Duque de Caxias Norte, 225, Pirassununga, Brazil
Natalia Cristina da Silva
Departamento de Engenharia de Alimentos, Faculdade de Zootecnia e Engenharia de Alimentos, Universidade de São Paulo, Rua Duque de Caxias Norte 225, Pirassununga, SP, Brazil; Postgraduate Programme in Materials Science and Engineering, University of São Paulo, USP/ FZEA, Av. Duque de Caxias Norte, 225, Pirassununga, Brazil
Adil Denizli
Department of Chemistry, Hacettepe University, Beytepe, Ankara, Turkey
Ankita Gupta
Institute of Environment & Sustainable Development Banaras Hindu University Varanasi, Uttar Pradesh, India
Mani Pujitha Illa
Cellulose and composites group, Department of Materials Science and Metallurgical Engineering, IIT Hyderabad, Telangana, India
Sapna Jain
Department of Chemistry, School of Engineering, University of Petroleum and Energy Studies, Dehradun, Uttarakhand, India
Shruti Jha
Sardar Vallabhbhai National Institute of Technology, Surat, Gujarat, India
Swaminathan Jiji
DRDO-BU Center for Life Sciences, Bharathiar University Campus, Coimbatore, Tamil Nadu, India
Krishna Kadirvelu
DRDO-BU Center for Life Sciences, Bharathiar University Campus, Coimbatore, Tamil Nadu, India
Mudrika Khandelwal
Cellulose and composites group, Department of Materials Science and Metallurgical Engineering, IIT Hyderabad, Telangana, India
Sanjeev Kumar
Department of Chemistry, School of Engineering, University of Petroleum and Energy Studies, Dehradun, Uttarakhand, India
Vibhanshu Kumar
Department of Water Engineering and Management, Central University of Jharkhand, Jharkhand, India
Himanshu Kumar
Department of Water Engineering and Management, Central University of Jharkhand, Jharkhand, India
Bhawna Yadav Lamba
Department of Chemistry, School of Engineering, University of Petroleum and Energy Studies, Dehradun, Uttarakhand, India
Kannan Maharajan
Biology Institute, Qilu University of Technology (Shandong Academy of Sciences), Jinan, Shandong Province, China
Santosh Kr. Mishra
Department of Production Engineering, National Institute of Technology Tiruchirappalli, Tamil Nadu, India
Mamata Mohapatra
CSIR-Institute of Minerals and Materials Technology, Bhubaneswar, Odisha, India; Academy of Scientific & Innovative Research, New Delhi, India
Aarohi Natu
School of Pharmacy, National Forensic Sciences University (Ministry of Home affairs, GOI), Gandhinagar, Gujarat, India
Nadia Obrownick Okamoto-Schalch
Departamento de Engenharia de Alimentos, Faculdade de Zootecnia e Engenharia de Alimentos, Universidade de São Paulo, Rua Duque de Caxias Norte 225, Pirassununga, SP, Brazil; Postgraduate Programme in Materials Science and Engineering, University of São Paulo, USP/ FZEA, Av. Duque de Caxias Norte, 225, Pirassununga, Brazil
Ramesh Oraon
Department of Nanoscience and Technology, Central University of Jharkhand, Brambe, Ranchi, Jharkhand, India
Arun. K. Padhy
Department of Chemistry, Central University of Jharkhand, Brambe, Ranchi, Jharkhand, India
Bansari Parikh
School of Pharmacy, National Forensic Sciences University (Ministry of Home affairs, GOI), Gandhinagar, Gujarat, India
Garvita Parikh
School of Pharmacy, National Forensic Sciences University (Ministry of Home affairs, GOI), Gandhinagar, Gujarat, India
Işık Perçin
Department of Biology, Hacettepe University, Beytepe, Ankara, Turkey
Prajesh Prajapati
School of Pharmacy, National Forensic Sciences University, Gandhinagar, Gujarat, India
Benjamin Raj
CSIR-Institute of Minerals and Materials Technology, Bhubaneswar, Odisha, India
Deepak Rawtani
School of Pharmacy, National Forensic Sciences University (Ministry of Home Affairs, GOI), Gandhinagar, Gujarat, India
Yulianti Sampora
Research Center for Chemistry – Indonesian Institute of Sciences (LIPI), Kawasan PUSPIPTEK, Serpong South Tangerang, Indonesia
Syed Saquib
Institute of Environment & Sustainable Development Banaras Hindu University Varanasi, Uttar Pradesh, India
Athanasia Amanda Septevani
Research Center for Chemistry – Indonesian Institute of Sciences (LIPI), Kawasan PUSPIPTEK, Serpong South Tangerang, Indonesia
Archana Singh
School of Engineering and Technology, National Forensic Sciences University (Ministry of Home Affairs, GOI), Gandhinagar, Gujarat, India
Gurudatta Singh
Institute of Environment & Sustainable Development Banaras Hindu University Varanasi, Uttar Pradesh, India
Purushottam Kumar Singh
Department of Mechanical Engineering, BIT Sindri, Jharkhand, India
P. R Sreeraj
Department of Production Engineering, National Institute of Technology Tiruchirappalli, Tamil Nadu, India
Swati
Department of Botany, Institute of Science, Banaras Hindu University Varanasi, Uttar Pradesh, India
Emel Tamahkar
Department of Bioengineering, Bursa Technical University, Bursa, Turkey
Anamika Shalini Tirkey
Department of Geoinformatics, School of Natural Resource Management, Central University of Jharkhand, Brambe, Ranchi, Jharkhand, India
Aykut Arif Topçu
Department of Medical Services and Techniques, Aksaray University, Aksaray, Turkey
Milena Martelli Tosi
Departamento de Engenharia de Alimentos, Faculdade de Zootecnia e Engenharia de Alimentos, Universidade de São Paulo, Rua Duque de Caxias Norte 225, Pirassununga, SP, Brazil; Postgraduate Programme in Materials Science and Engineering, University of São Paulo, USP/ FZEA, Av. Duque de Caxias Norte, 225, Pirassununga, Brazil
Riddhi Trivedi
School of Pharmacy, National Forensic Sciences University, Gandhinagar, Gujarat, India
Shashikant Shivaji Vhatkar
Department of Nanoscience and Technology, Central University of Jharkhand, Brambe, Ranchi, Jharkhand, India
Bacterial cellulose nanofibers for separation, drug delivery, wound dressing, and tissue engineering applications
Emel Tamahkara, Aykut Arif Topçub, Işık Perçinc, Sevgi Aslıyüced, Adil Denizlid
aDepartment of Bioengineering, Bursa Technical University, Bursa, Turkey
bDepartment of Medical Services and Techniques, Aksaray University, Aksaray, Turkey
cDepartment of Biology, Hacettepe University, Beytepe, Ankara, Turkey
dDepartment of Chemistry, Hacettepe University, Beytepe, Ankara, Turkey
1.1 Introduction
Bacterial cellulose (BC) nanofibers can be produced by bacteria, plants, and algae. Among them the most utilized source is Acetobacter xylinum due to the production efficiency. Although BC has similar chemical structure with plant cellulose, BC attracted tremendous interest with its high purity devoid of hemicellulose and lignin thus, avoiding the purification cost [1]. BC presents many advantages over plant cellulose regarding the production expenses. Cost-efficient bacterial culture medium is utilized in the preparation of BC. Also the synthesis is simple and requires no special equipment and any harsh chemicals [2]. BC is synthesized as a pellicle composed of unoriented fibers of cellulose chains resulting in 3D nanofibrous network. These nanofibers are approximately 50–100 nm in diameter. BC is formed via beta glycosidic bonds, and glucan chains are linked through intermolecular and intramolecular hydrogen bonds [3]
BC is one of the mostly used biomaterial for academic, industrial, and pharmaceutical areas designed as drug delivery vehicle, wound dressing, separation medium, and tissue engineering scaffold. BC presents excellent potential for these areas with its high water absorption capacity, high porosity, high purity, and high mechanical strength. Also BC can be fabricated in any size and shape due to its in-situ moldability properties suggesting great applicability for diverse applications [4]. Thus, the aim of this chapter is focused on the recent developments of BC in these application areas.
1.2 Support as separation medium
As BC nanofibers exhibit nanoporous structure, biocompatibility, high water-holding capability, and chemical stability, they have big potential to be used for separation and removal of many compounds. BC nanofibers are attractive materials to be prepared
https://doi.org/10.1016/B978-0-12-823963-6.00004-1
as a separation media for the removal of environmental pollutants and purification of biologically important molecules. BC nanofibers are resistant to different environmental conditions and have high surface area with nanofibrous network [5]. Separation processes based on many intermolecular interactions need to be selective and specific to target molecules. Thus, modifications of BC using in separation applications have great importance for preparation of effective adsorbents [6]. BC nanofibers with their unique properties can be functionalized by many different chemical methods to show good bio-affinity properties. BC nanofibers also can be prepared by combining natural and synthetic polymers, thus changing surface and bulk properties.
Protein-specific BC-based nanofibers were prepared by Denizli and coworkers. Göktürk et al. designed human serum albumin imprinted composite BC nanofibers for proteomic applications. Molecular imprinting is a promising technique to obtain selective binding sites formed via polymerization around the template molecule with further extraction of the template from the polymeric material. Molecularly imprinted polymers (MIP) show great potential with high selectivity. As albumin is a high-abundance protein, they fabricated albumin imprinted composite BC nanofibers via metal ion coordination interactions using N-methacryloyl-(L)-histidine methyl ester (MAH) and Cu(II) ions (Fig. 1.1A).They tested selectivity of albumin imprinted BC by using human transferrin and myoglobin as competitive agents. Furthermore, the depletion of human serum albumin from artificial human plasma was performed by albumin-imprinted
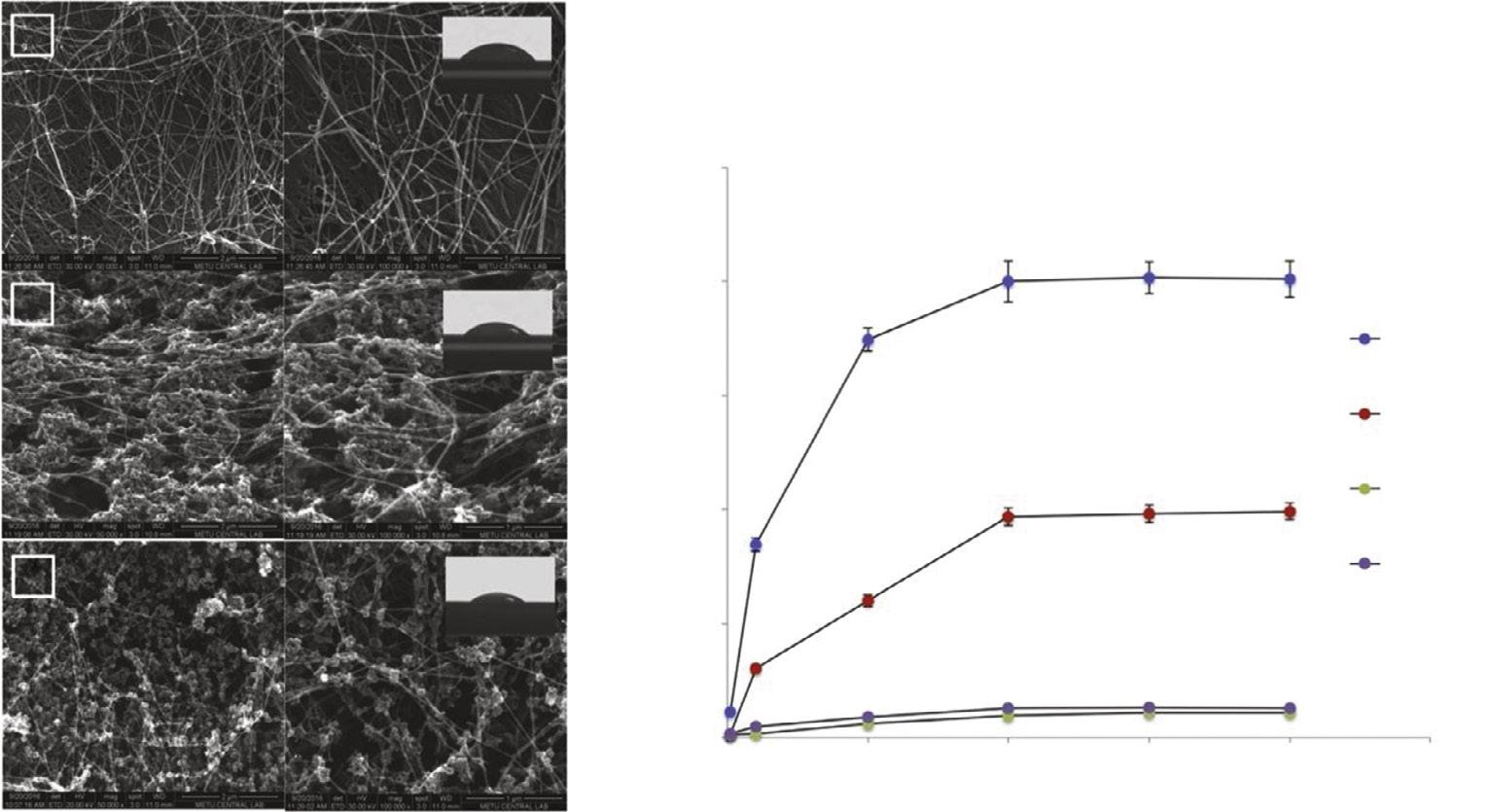
Fig. 1.1 (A) Scanning electron microscope images of BC (top), molecularly imprinted BC (middle) and nonimprinted BC (bottom). (B) The adsorption capacity of hemoglobin onto MIP-BC and NIP-BC. (A) Adapted with permission from [7], (B) Adapted with permission from [10].
BC nanofibers and efficiency of these BC nanofibers was shown with SDSPAGE and 2D gel electrophoresis analyses [7]. Tamahkar et al. aimed to deplete human serum albumin from human serum to provide easier study of low-abundance proteins, which have potential value for clinical diagnosis, for proteomic applications. For this purpose, they produced cibacron blue F3GA (CB) bound BC nanofibers. Specific surface area of CB-bound BC nanofibers was obtained as 914 m2/g and albumin binding capacity of CB-bound BC nanofibers was found as 1800 mg/g. Elution of albumin from CB-bound BC nanofibers was achieved by 1 M NaCl and reusability was shown. In addition, albumin was purified from human serum successfully by using CB-bound BC nanofibers [8]. Saylan et al. developed lysozyme-imprinted BC (Lyz-MIP/BC) nanofibers for recognition of lysozyme. They applied surface molecular imprinting method by imprinting lysozyme on the surface of BC nanofibers in the presence of metal ions. The maximum lysozyme adsorption capacity of lysozyme-imprinted BC nanofibers obtained was 71.1 mg/g. Bovine serum albumin and cytochrome c were chosen as competitive proteins in this study and lysozyme-imprinted BC nanofibers showed high selectivity for lysozyme against these proteins. Reusability and high characteristic properties of lysozyme-imprinted BC nanofibers were also shown by authors [9]. Bakhshpour et al. fabricated a hemoglobin-imprinted film onto BC nanofibers. They preferred surface imprinting method by taking advantage of metal ion coordination interactions using MAH and copper ions. Hemoglobin was selectively purified from hemolysate using hemoglobin surface–imprinted BC nanofibers. Authors indicated structural and geometrical complementarity between recognition sites and template hemoglobin molecules by performing selectivity studies using cytochrome c, lysozyme, bovine serum albumin, and myoglobin. Maximum hemoglobin binding capacity of hemoglobin-imprinted BC nanofibers was 208.39 mg/g (Fig. 1.1B) [10]. Tamahkar et al. designed surface-imprinted BC nanofibers for cytochrome c purification. They used metal ion coordination interactions using copper and MAH as a functional monomer orienting cytochrome c molecules. After determining optimum experimental conditions they have obtained high recognition capacity. Authors also extracted cytochrome c from rat liver extract and eluted cytochrome c from cytochrome c–imprinted BC nanofibers successfully. Thus, they presented a novel, cheap, and simple procedure for efficient purification of cytochrome c [11]. Another study from Denizli and coworkers was reported for BC nanofibers as a protein adsorbent. 4-vinylimidazole (VIm) and a household monomer, MAH, were used as metal-chelating monomers. These monomers were complexed with Cu(II) and Ni(II) metal ions for carrying out metal ion coordination interactions with proteins. They have chosen hemoglobin as a model protein and the maximum hemoglobin binding capacity of the modified BC nanofibers was found as 47.40 mg/g [12]
The removal of some toxic substances from environment was also achieved by using BC nanofibers. Derazshamshir et al. used N-methacryloyl-(L)-amido phenylalanine as a
functional monomer for the preparation of phenol-imprinted BC nanofibers by surface imprinting approach. They have applied surface-imprinted BC (BC-MIP) nanofibers to real wastewater samples from Ergene basin in Turkey and evaluated phenol removal efficiency of BC-MIP nanofibers. 2-chlorophenol, 4-chlorophenol and 2,4-dichlorophenol were used as computing agents and phenol removal efficiency was obtained as 97% [13].
Hou et al. presented functional BC membranes for water/oil separation for a solution to oil spill accidents that cause serious environmental pollution. They modified BC nanofibers by hydrolyzing of tetraethoxysilane and obtained BC@SiO2 networks that were capable of separation of oil/water emulsions with high separation efficiency. Thus, they developed a promising potential wastewater treatment tool due to renewable and biodegradable nature of BC nanofibers [14]. Jin et al. prepared polyethyleneimine-BC nanofibers for the removal of copper and lead ions from aqueous solutions. Maximum binding capacities of polyethyleneimine-BC for copper and lead ions were obtained as 141 and 148 mg/g, respectively. Adsorption processes of these metals reached equilibrium in 30–60 min and were fitted with the Freundlich model. They demonstrated that polyethyleneimine-BC nanofibers are reusable and good alternative tools as bioadsorbents for heavy metal removal from wastewater [15]. Yang et al. prepared an efficient adsorbent by in-situ functionalization of poly(m-phenylenediamine) nanoparticles (NPs) on BC. Optimized poly(m-phenylenediamine) BC showed the Langmuir adsorption model with adsorption capacity of 434.78 mg/g for Cr(VI) ions. Authors observed high stabilization of poly(m-phenylenediamine) on BC and reusability of poly(m-phenylenediamine) BC nanofibers [16]. Shoukat et al. modified BC nanofibers with titanium oxide (TiO2) and prepared novel TiO2-BC nanofibers for the removal of lead ions from aqueous solutions. A concentration of 100 mg/L of lead was removed with 90% efficiency by TiO2-BC nanofibers in 120 min at pH 7.0 at room temperature. TiO2-BC nanofibers could be used three times without any decrease in lead adsorption capacity. Authors suggested TiO2-BC nanofibers as an effective, stable, and reusable tool for the removal of lead from wastewater samples [17]. Chen et al. prepared attapulgite/chitosan (ATP/Chi) composite with BC nanofibers exhibiting high binding capacity for metal ions such as lead, copper, chromium, and anionic organic dyes including Congo red. ATP/Chi BC nanofibers were reusable after five adsorption-desorption cycles. ATP/ Chi BC nanofibers with their multifunctional properties were effective tools to remove pollutants from environmental wastewater [18]. Hu et al. fabricated an organic–inorganic composite material combining BC and Ca-montmorillonite (Ca-MMT) to remove methylene blue (MB) and tetracycline (TC) from complex wastewater. Adsorption characteristics were well fitted with the Langmuir model and were suitable for a pseudo-second-order model. The removal of both MB and TC was successfully achieved even in the presence of other coexisting molecules. Low-cost Ca-MMT composite BC nanofibers were effectively reusable. The adsorption amount of the binary system
containing MB and TC was also investigated and the images of the mixture solutions before and after treatment with the prepared adsorbent demonstrated great separation performance (Fig. 1.2) [19]. Wan et al. prepared bacteria containing BC nanofibers with a novel approach keeping bacteria in the nanofiber network by skipping bacteria removal process. Bacteria containing composite BC nanofibers showed much higher adsorption capacities for lead, copper, nickel, and chromium ions compared to pure BC nanofibers. Authors explain higher adsorption capacity with the presence of functional groups in bacteria such as amide providing coordination interactions. Thus, a simpler and cheaper strategy for producing a novel BC-based adsorbent with high heavy metal binding capacity was applied [20]. Ashour et al. designed flexible BC nanofibers by immobilizing metal organic framework (MOF) containing Fe(III) 1,3,5-benzenetricarboxylate) on BC nanofibers by in-situ one-pot green method. MOFs are very popular in recent years and can be modified to be selective adsorbents. The novel MOF BC nanocomposites were used for the removal of arsenic and Rhodamine B from aqueous solutions with binding capacities of 4.81 and 2.77 mg/g, respectively [21]
1.3 Wound dressings
Wound that is a defect in the structure and function of the skin can be caused by trauma, burn, diabetes, etc. Wound healing has four stages of hemostasis, inflammation, proliferation, and tissue remodeling [22]. Hemostasis, which is the first phase of the healing process, starts with the injury and during this phase bleeding is stopped with the clotting system. Inflammation occurs to prepare the wound bed and to remove the bacteria. During proliferation, granulation tissue covers the wound site and keratinocytes and fibroblasts migrate. Finally, the new tissue is remodeled. It is evaluated that 20–60 million people will endure chronic wounds by 2026 in the world [23]. An ideal wound dressing should provide an adequate moist environment for an effective wound healing process as humidity is required to maintain the healing. The properties of water retention capacity of the wound dressings affect substantially the healing to eliminate the excess liquid from the wound area. Also wound dressing with humid environment can act as a barrier against infections, and can be displaced easily on demand. Furthermore wound dressing having moderate water absorption amount can serve as a drug delivery vehicle to facilitate the healing [24].
BC with its unique characteristics such as high mechanical strength, high flexibility, high water-retention capacity, permeability for gas transfer, and high biocompatibility is evaluated as an ideal wound dressing material [25]. BC has been applied as a wound dressing material since 1980s [26]. There exist many commercial BC-based wound dressings namely Biofill that is Bionext, Membracell, and Xcell applied successfully for ulcers and burns. Biofill produced by a Brazilian company Biofill Produtos Bioetecnologicos is the first commercial BC-based wound dressing and is applied to burns, chronic skin ulcers. This company also manufactures Bionext and Membracell
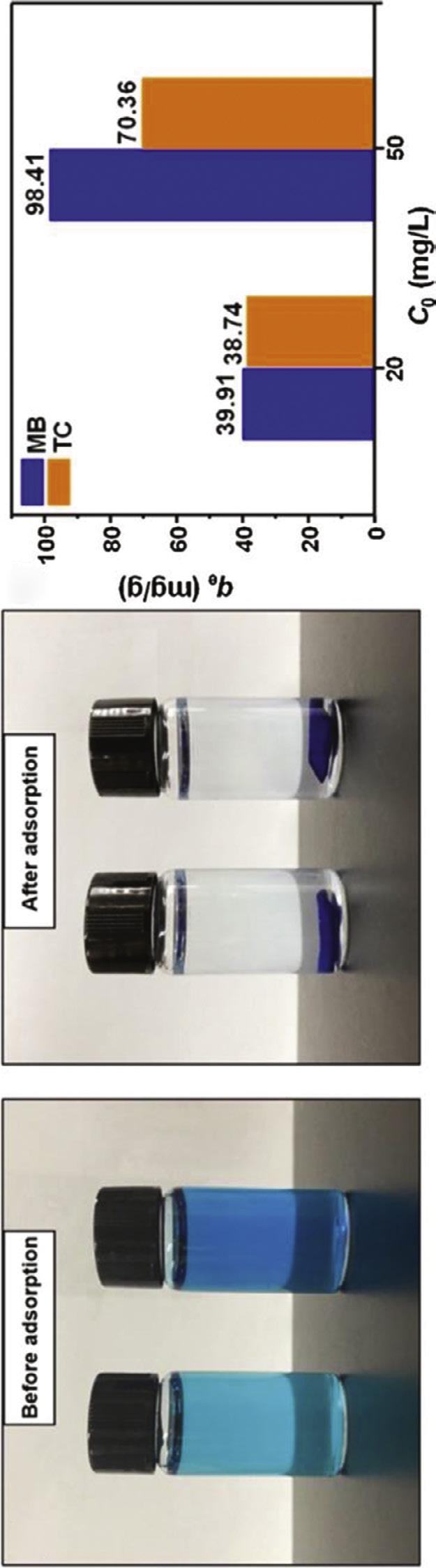
Fig. 1.2 Photographs of the MB and TC mixture solutions before and after the treatment with BC-based adsorbent. (A) A 20 mg/mL MB-TC binary solution (left), 50 mg/mL binary solution (right) before adsorption, (B) after adsorption. (C) The maximum adsorption amounts of MB and TC onto BC-based adsorbent. Adapted with the permission from [19] .
for burns and chronic ulcers. Xylos Co. productized Xcell in 1996 as a Food and Drug Administration approved wound dressing having two significant features of absorption of exudate and holding the wound moist [27].
BC ensures efficient wound healing due to high water retention capability. The unbonded water molecules entrapped in the pores of BC function for the hydration [28]. BC with macropores and 3D morphological structure exhibits a suitable platform for the cell proliferation. This nanofibrous network structure also enables gas transfer throughout the membrane. BC can be utilized safely without any toxicity owing to its high biocompatibility. Solway et al. focused on the comparison of BC and commercial wound dressing namely Xeroform in regard to wound healing rate of diabetic foot ulcers. The rate of wound closure of BC was 1.7 times higher than that of Xeroform. BC provided higher water-holding capacity and water removal with high mechanical strength. It was mentioned that the pore size and the nanofibrous network structure of BC enable to start hemostasis and facilitate the formation of granulation tissue and epithelization [29]
To control the humidity of the nanofibers, the incorporation of polymers as a second component throughout BC matrix can be conducted to enhance the waterholding capacity. The decrease of the time period of dehydration decreases the exchange frequency, thus reduces the treatment costs. Sulaeva et al. developed BC/alginate films loaded with poly(hexamethylene biguanide) hydrochloride (PHMB) that is a cationic antimicrobial agent. BC was immersed in the alginate solution of different concentrations with subsequent cross-linking with CaCl2. The feasibility of the large-scale production of BC/alginate membranes loaded with PHMB was also examined and the time required for the preparation of sterilized, and packaged novel BC-based wound dressing was reported as 4 days [30].
Transparency is an important concern for wound dressings as transparent materials enable to inform about the status of the wound and to monitor the healing without detaching of the dressing. Thus, transparent dressings provide patient comfort and efficient healing without interruption and any hospitalization cost. Tabaii et al. prepared transparent antibacterial BC-based wound dressings with silver NPs (AgNPs). The in-situ incorporation of AgNPs was conducted using sodium tripolyphosphate (TPP) or sodium hydroxide (NaOH) as reducing agents. The transparent BC-based wound dressings impregnated with AgNPs were formed when utilizing TPP (2%) and NaOH (0.04%). The resultant dressings showed excellent antibacterial activity against Escherichia coli and Staphylococcus aureus with ease of wound inspection of high transparency [31]. BC was applied as 3D wound dressing via in-situ modification with polyvinyl alcohol. It was prepared utilizing oxygen-permeable mold in the shape of a glove [32]
The impregnation of metallic NPs such as silver, zinc oxide, and copper into BC matrix has attracted wide interest approach to obtain antibacterial dressing. These metallic NPs present great antimicrobial activity due to their unique nanoscale characteristics.
Metallic NPs inhibit microbes via disarrangement of cell wall and production of reactive oxygen species inside the organism [33]. AgNPs with broad-spectrum antimicrobial features yield high antibacterial activity due to high surface area and widely utilized to fabricate wound dressings. Silver metal changes to silver ion under physiological conditions and exhibit antibacterial performance by binding the thiol groups of enzymes and proteins thus adjusting the bacterial metabolic pathways. Silver ion displays no toxicity to human cells except high concentrations of Ag+ as it causes DNA damage to mammalian cells. BC represents an efficient support material by means of unique morphological character for the uniform distribution of AgNPs to avoid the aggregation of the NPs. There exist many reports about the preparation of AgNP-integrated BC using NaBH4, triethanolamine, hydrazine, TEMPO (2,2,6,6-Tetramethylpiperidin-1-yl)oxyl), UV-radiation [34,35]. However, these reducing agents can cause toxicity concerns. Recently, the development of AgNP-incorporated BC membranes via a green route is gaining much attention. The choice of reducing agent, solvent, and stabilizer is the key parameters for green approach. Gupta et al. developed BC-based wound dressing with the integration of AgNP using a curcumin-cyclodextrin complex as a reducing agent. The AgNPs were produced with the curcumin-cyclodextrin complex with subsequent integration into BC. The prepared AgNP-integrated BC dressings exhibited a high survival rate due to MTT (3-(4,5-dimethylthiazol-2-yl)-2,5-diphenyltetrazolium bromide) assay using Panc 1 (human pancreatic ductal adenocarcinoma), U251 (human glioblastoma), and MSTO (human mesothelioma). Also the AgNP-integrated BC hydrogels displayed high any antibacterial activity against Pseudomonas aeruginosa, S. aureus, and Candida auris, while cyclodextrin-integrated BC and BC hydrogels as controls demonstrated antibacterial functionality [36]. The strength of the interactions between BC and AgNPs has great importance as the poor binding occurred between BC and the AgNPs can lead to fast release causing toxic effects. Thus, the design of the AgNPsincorporated BC dressings should ensure the release of silver ions in a controlled manner. Wu et al. reported the release profile of Ag+ from AgNP-BC dressings prepared with Tollen’s reagent using BC as a nanoreactor (Fig. 1.3). The resultant dressings showed controlled release of silver ions in comparison with the control groups which were neat BC, AgNO3-BC (prepared via immersion of BC into silver nitrate solution), and AgNP-BC (prepared via immersion of BC into the solution of AgNPs). This proves the strong attachment of AgNPs onto BC matrix thus preventing the uncontrolled release of AgNPs [37]. Khalid et al. prepared zinc-oxide NPs and then added them into BC structure with subsequent freeze-drying to fabricate BC/ZnO dressings. BC/ZnO dressings showed 90% bacterial inhibition on average for wound pathogens proving the bactericidal effect of the resultant wound dressings with zinc-oxide NPs. Higher wound healing performance and tissue regeneration was achieved with BC/ZnO dressings rather than that of BC due to participation of zinc during healing process [38]. In addition, copper NPs were incorporated into BC network structure to yield an antibacterial wound dressing via chemical reduction and hydrothermal method [39,40].
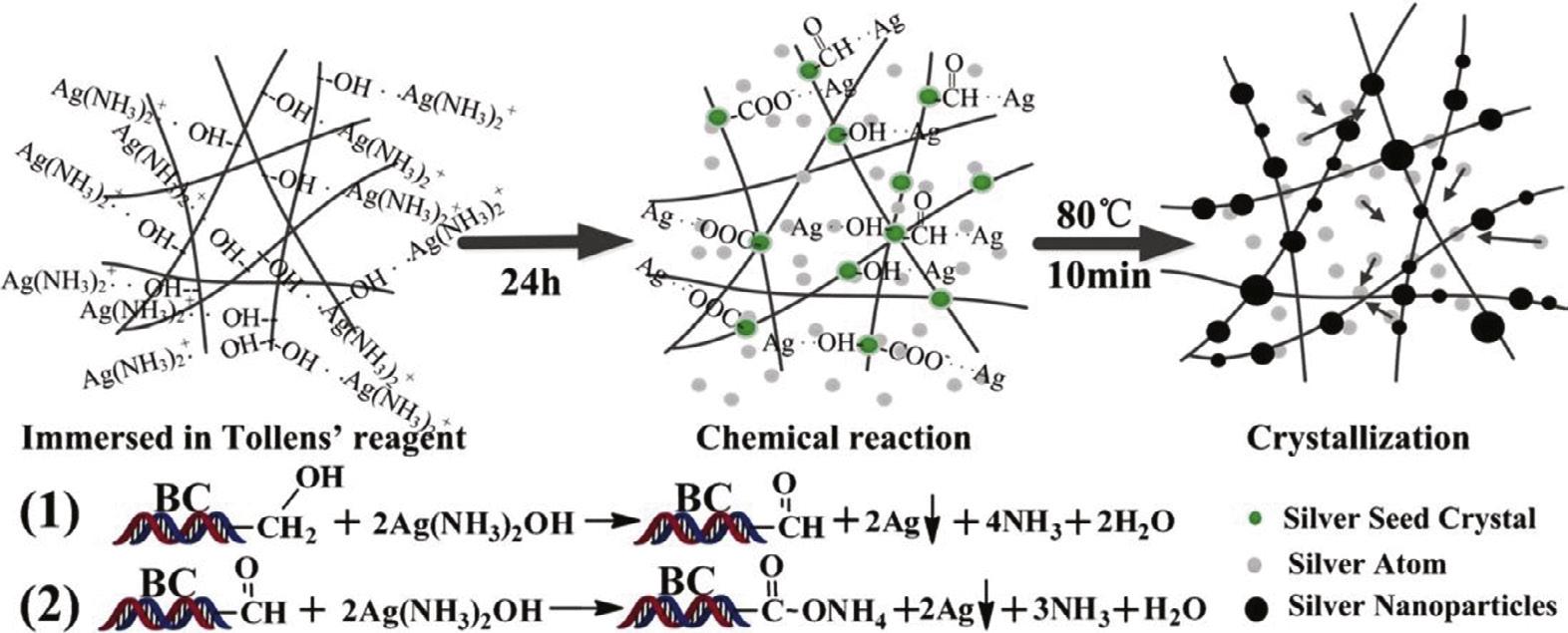
However silver can be integrated in the form of silver sulfadiazine (SSD) that is a common, broad-spectrum topical antibacterial agent utilized widely in the burn treatment. To prevent the cytotoxic side effects of SSD, BC-based wound dressings were formed with sodium alginate. SSD-incorporated BC/alginate hydrogels prepared in a simple route showed good biocompatibility on human embryonic kidney cells and great antibacterial performance againts E. coli, S. aureus, and Candida albicans [41]. In another study, SSD nano- (282 nm) and micro- (2149 nm) particles were synthesized and further integrated with a BC matrix for burn treatment. Owing to antibacterial activity tests, SSD-incorporated BC membranes demonstrated excellent antibacterial performance against Gram-positive and Gram-negative bacteria and high healing rate [42].
Some features of BC can be altered to improve the efficiency of healing performance. For this context, the incorporation of antimicrobial agent into the BC matrix is one of the general approaches to gain antibacterial functionality against the common bacteria that cause infections in the wound region. Amoxicillin is one of the common broad-spectrum antibiotics utilized for the infection. However its usage is restricted owing to the tendency to lose its function easily. Thus, grafting of the antibacterial agent becomes a good alternative. For the grafting of amoxicillin onto BC, first BC was modified with (3-aminopropyl) triethoxysilane and then amoxicillin was covalently bound with EDC/NHS (1-ethyl-3-(3-dimethylaminopropyl)carbodiimide/Nhydroxysuccinimide). Amoxicillin-grafted BC displayed improved antibacterial performance in comparison with neat BC. Also amoxicillin-grafted BC prepared with the drug concentration of 1 mg/mL showed a high wound closure rate of 45% after 3 days of treatment, while bare wound and BC as controls demonstrated 20% [43] Qiu et al. developed BC-based wound dressings with the incorporation of vaccarin, a major flavonoid, which promotes the endothelial cell proliferation. It was determined that vaccarin-loaded BC membranes showed better healing performance due to rapid epithelization and regeneration [44]
Fig. 1.3 The schematic presentation of the preparation of AgNP-BC. Adapted the permission from [37]
Chi was also utilized to form interpenetrating network within BC network structure to gain antibacterial functionality. Lin et al. prepared BC/Chi membranes by immersion of BC into Chi solution with subsequent lyophilization. After the incorporation of Chi through BC network, BC/Chi membranes demonstrated great antibacterial activity against E. coli and S. aureus. Also epithelization of the BC/Chi membranes was reported faster than that of BC due to histological evaluations [45]. Doping of Chi onto BC also provides controlled release of antibiotics by altering the dimensions of the pore size of BC network besides antimicrobial activity and healing ability. Cacicedo et al. prepared Chi-impregnated BC hydrogels (BC/Chi) with ciprofloxacin loading for wound management. BC/Chi showed better water vapor transmission rate (WVTR) compared to that of BC. WVTR is one of the significant parameters indicating the capability of the material to keep the wound moist. The delivery of ciprofloxacin from BC/Chi reached an equilibrium at approximately 70% after 6 h offering a fast release for bacterial invasion at the beginning of the treatment. BC/Chi films exhibited high antibacterial activity after ciprofloxacin loading because of the synergistic effect of Chi with the antibiotic [46]. Furthermore, antimicrobial character was gained throughout the BC via covalent attachment of aminoalkyl groups [47].
1.4 Drug delivery vehicle
When the history of drug delivery systems (DDSs) is examined, DDS could be categorized into three stages; in the first generation between 1950 and 1980, researchers tried to understand the releasing mechanisms on DDS and focused on developing the oral and transdermal-based DDS, which were just capable of releasing the drugs in short periods. In the second generation between 1980 and 2010, nanotechnology-based DDS such as smart polymers and hydrogels were developed to use in DDS and the new drug carriers were able to release the drugs by changing the environmental conditions such as pH and temperature. Besides, the protein and peptide releasing studies in long periods were introduced in the second generation by using the other carriers and the first implant; Zoladex Depot was used to deliver goserelin acetate in 1989. The third generation between 2010 and 2040, called modulated delivery systems aims to release drugs to tumors, gene delivery, develop long-term delivery systems, and investigate in the suitable formulations of drugs for clinical trials [48]. DDS aims to transport the drugs needed by organs, cells and tissues at therapeutic concentrations by using suitable carriers. Moreover, this approach brings along various advantages such as increasing the solubility of the drugs, enhancing pharmaceutical activities, maintaining the chemically active agents, and reducing not only the drug aggregations but side effects, so on. However, if aimed to design an effective DDS, some parameters such as the releasing mechanism and releasing kinetic of a selected drug and its carrier selection should be carefully optimized [49].
BC nanofibers having superior properties such as high surface area, high porosity, mechanical properties, and mimicking the extra cellular matrix attracted much attention in drug delivery [50]. Tamahkar et al. aimed to extend the release of gentamicin sulfate by molecular imprinting technique (MIT), allowing designing the tailor-made receptors capable of selectively recognizing the template molecules. For this purpose, gentamicin sulfate–imprinted microparticles were created on BC surface by using methacrylic acid as a functional monomer and methylene bisacrylamide (MBAAm) as a cross-linker. The release of gentamicin sulfate studies were investigated in in-vitro drug studies. In the light of the experimental results; the creation of shape-memory effects based on the specific interactions between the functional monomer and drug by using with MIT can not only enhance the drug loading capacity but also extend the releasing of gentamicin sulfate when compared to the non-imprinted BC fiber (NIP) and gentamicin sulfate–imprinted BC fiber (MIP). The loading capacity of gentamicin sulfate on NIP was nearly five fold lower than MIP and gentamicin sulfate–imprinted BC fibers (MIPS) released the drugs within 48 h, whereas NIP released gentamicin sulfate in just 8 h; hence, imprinting cavities could enhance the loading capacity and release of drug of interest by adjusting the experimental conditions [51].
The performance of BC was aimed to hybridize with chitin to yield antibacterial function, and curcumin were formed in-situ to obtain the delivery in a controlled manner. The squid pen was chosen as a natural source of chitin, and before the formation of chitin nanofibers, chitin was isolated from its natural source by ultrasonication, after that the supernatant of the isolated solution was centrifuged and lyophilized, respectively. Before the hybridization of the chitin with BC-based nanofibers, a bacteria, Gluconacetobacter xylinus, was used for producing of BC pellicles with the suitable growth media, and the alkaline treatment method was applied to fabricate nanofibrillated BC films. After that, to fabricate the hybrid nanofibrillated BC-Chi nanofiber films, the same procedure was used for the formation of the BC, and then the curcumin particles in the ranges of micro- and nano-sizes were created, on which the desired surfaces were formed by the precipitation method. According to the experimental results, the incorporation of chitin could enhance the strength of the fabricated nanofibers, and interestingly the curcumin particles on the hybrid nanofiber surfaces could use an indicator of boric acid that is unsafe food additives, harmful for human health; moreover, the hybrid nanofibers gain the antibacterial properties thanks to the formation of the curcumin particles on their surfaces [52]
In the next study, chlorhexidine (CHX), a widely used model drug, was chosen because of its safety and antiseptic property. In this sense, the researchers investigated the in-vitro release profile of CHX and tested its antimicrobial properties against three different microorganisms. For this goal, the BC was produced by growing Komagataeibacter hansenii and the fibers were oxidized with the use of different concentrations of NaIO4, following that, CHX was loaded on drugs carrier by using different approaches. In the