Adaptive Phytoremediation Practices
Resilience to Climate Change
Vimal Chandra Pandey
Department of Environmental Science, Babasaheb Bhimrao Ambedkar University, Lucknow, India
Gordana Gajić
Department of Ecology, Institute for Biological Research “Sinisa Stankovic”, National Institute of Republic of Serbia, University of Belgrade, Belgrade, Serbia
Pallavi Sharma
School of Environment and Sustainable Development, Central University of Gujarat, Gandhinagar, India
Madhumita Roy
Department of Microbiology, Bose Institute, Kolkata, India
Elsevier
Radarweg 29, PO Box 211, 1000 AE Amsterdam, Netherlands
The Boulevard, Langford Lane, Kidlington, Oxford OX5 1GB, United Kingdom 50 Hampshire Street, 5th Floor, Cambridge, MA 02139, United States
Copyright © 2022 Elsevier Inc. All rights reserved.
No part of this publication may be reproduced or transmitted in any form or by any means, electronic or mechanical, including photocopying, recording, or any information storage and retrieval system, without permission in writing from the publisher. Details on how to seek permission, further information about the Publisher’s permissions policies and our arrangements with organizations such as the Copyright Clearance Center and the Copyright Licensing Agency, can be found at our website: www.elsevier.com/ permissions.
This book and the individual contributions contained in it are protected under copyright by the Publisher (other than as may be noted herein).
Notices
Knowledge and best practice in this field are constantly changing. As new research and experience broaden our understanding, changes in research methods, professional practices, or medical treatment may become necessary.
Practitioners and researchers must always rely on their own experience and knowledge in evaluating and using any information, methods, compounds, or experiments described herein. In using such information or methods they should be mindful of their own safety and the safety of others, including parties for whom they have a professional responsibility.
To the fullest extent of the law, neither the Publisher nor the authors, contributors, or editors, assume any liability for any injury and/or damage to persons or property as a matter of products liability, negligence or otherwise, or from any use or operation of any methods, products, instructions, or ideas contained in the material herein.
Library of Congress Cataloging-in-Publication Data
A catalog record for this book is available from the Library of Congress
British Library Cataloguing-in-Publication Data
A catalogue record for this book is available from the British Library
ISBN: 978-0-12-823831-8
For information on all Elsevier publications visit our website at https://www.elsevier.com/books-and-journals
Publisher: Candice G. Janco
Acquisitions Editor: Marisa LaFleur
Editorial Project Manager: Aleksandra Packowska
Production Project Manager: Joy Christel Neumarin
Honest Thangiah
Cover Designer: Vicky Pearson Esser
Typeset by STRAIVE, India
Preface
Adaptive Phytoremediation Practices: Resilience to Climate Change presents phytoremediation practices in the scenario of changing climate and plant responses to stress. The book provides a brief overview of different aspects of adaptive phytoremediation practice and responses of plants to climatic stressors and pollution. It presents the current state of research on structural and functional characteristics of resilient plants and advancements in the knowledge on lipidomics and designer plants that can be applied in agriculture and phytoremediation using gene editing tools such as the CRISPR/Cas 9 system. The book covers all aspects of soil and phytomanagement and offers prospects for the development of climate-resilient economic crops. It aims to provide knowledge on the bioeconomy of contaminated biomass, ecosystem services, and policy frameworks. Appropriate green remediation strategies are essential for climate, energy, agricultural, and food policy domains linking the environment, people, markets, assets, and finance to conserve natural resources and improve human health and well-being worldwide. This book will be ideal for students, researchers, environmentalists, ecological engineers, regulatory agencies, policy makers, and other stakeholders.
Chapter 1 provides general information about the impacts of climate change on plant growth and metal uptake together with plant-microbe interaction to help readers better understand the impacts of elevated CO2, temperature, and drought on plants. Chapter 2 covers plant responses to abiotic and biotic stresses, such as high temperatures, drought, salinity, cold, flood, heavy metals, and pests, highlighting plant tolerance. Chapter 3 elaborates the functional and structural responses of resilient plants to climate change and pollution with a focus on plant physiology, biochemistry, anatomy, and morphology. Chapter 4 provides a detailed overview of soil management using arbuscular mycorrhizae against drought and salinity stress and rhizobacteria and industrial and organic wastes in the management of degraded soils, with special emphasis on biofuel, fiber, aromatic essential oil, and fortified crop production and their role in phytoremediation. Chapter 5 focuses on phytoremediation strategies and ecosystem services for polluted sites through provisioning, regulating, and supporting services. A detailed review of biotechnological strategies for the generation of designer plants for climate-resilient phytoremediation involving the application of CRISPER for cold, salt, heat, and drought plant tolerance, and improvement of their yields, quality, and nutrition, is presented in Chapter 6. Making biomass from phytoremediation; detailed information on biochemical and thermochemical processes; the recovery of biodiesel, biogas, bioethanol, and dye from contaminated biomass; the conversion of biomass
Preface to bioelectricity; and making bioenergy from farm waste are covered in Chapter 7. Finally, Chapter 8 describes the status of remediation and policy implications, climate smart agriculture, and phytoremediation all of which aim to create a greener and sustainable economy for the protection of the environment and human health around the globe.
Vimal Chandra Pandey Gordana Gajić Pallavi Sharma Madhumita Roy
2 Global climate change
Climate changes before industrial revolution during the 1700s may be attributed to natural causes, including volcanic eruptions, alterations in solar energy, and natural variations in GHG such as carbon dioxide (CO2), water vapor, methane (CH4), ozone (O3), and nitrous oxides (NxO) (IPCC, 2013). However, natural causes singly cannot explain current climate changes. Warming from the mid of 20th century has been attributed mainly to human activities. Fossil fuel (coal, oil, and gas) burning for heat, electricity, and transportation and deforestation are leading sources of anthropogenic emissions of GHG. Other anthropogenic activities like fertilizer usage (nitrous oxide), production of livestock (CH4), and some industrial processes (fluorinated gases) also lead to GHG production. GHG absorb thermal infrared (IR) radiation, which is discharged by the surface of Earth and atmosphere and, in turn, emits IR radiation, with a substantial part of this energy used in warming the surface of Earth and lower atmosphere (Ledley et al., 1999). Increased GHG emissions can provoke extreme climate changes such as heat, floods, and droughts. Some GHGs such as CH4 can persist for up to decades, whereas CO2 stays for centuries to millennia (Zickfeld et al., 2017). The concentration of CO2, which is the earth’s main climate change contributor, has enhanced by > 40% due to various human activities since preindustrial times. The atmospheric global CO2 growth rate was around 0.6 ± 0.1 ppm year 1 during the 1960s. However, between 2009 and 2018, the rate of growth was 2.3 ppm year 1 . The CO2 was around 409.8 ± 0.1 ppm (global average) in year 2019, an increase of 2.5 ± 0.1 ppm compared to 2018. By this century end, atmospheric CO2 is expected to be around 700 ppm (Lindsey, 2020).
Rising CO2 can affect plant metabolism both directly and indirectly. CO2 has an important role in photosynthesis so it can directly affect photosynthesis. Indirectly, it can affect plant performance via its impact on temperature and drought (Dusenge et al., 2019). Increased CO2 concentration amplifies Earth’s greenhouse effect. Strong correlation between CO2 concentration and air temperature has been noted throughout glacial cycles in past numerous hundred thousand of years. When the CO2 concentration increases, temperature also goes up. Also, anthropogenic activity-induced climate change has also led to incidence and intensity of temperature extremes (Meehl et al., 2007; Seong et al., 2021). IPCC estimated that by the year 2100, an increase in temperature will be between 1.8°C and 4.0°C (IPCC, 2014). Heat waves or extreme temperature events are projected to become more intense, more frequent, and last longer than what is being currently observed (Meehl et al., 2007). Daily minimum temperatures are suggested to increase more quickly compared to daily maximum temperatures causing increased daily mean temperatures and a higher risk of extreme events, which can cause damaging effects on plant (Meehl et al., 2007). As temperature is among the key environmental factor controlling the plant growth and development
(Hatfield and Prueger, 2015), the responses of plant to temperature variations have become a major concern nowadays.
Inconsistency in temperature and occurrence of precipitation may lead to a rise in water scarcity. Therefore, due to global warming and change in climatic conditions, changes in distribution, the frequency, severity, and duration of drought are expected to increase in the future. For 21st century, a steady rise in aridity has been projected by Global climate models (Scheff and Frierson, 2015; Zarch et al., 2017; Greve et al., 2019). According to these projections, drylands will possibly expand due to surges in evaporative demand and a hydrological cycle having extended and more severe dry periods at the worldwide scale. Also, an enhancement in soil salt concentration and a reduction in water quality may lead to soil salinization, which may cause land degradation (Tomaz et al., 2020). River flood hazard may also increase owing to climate alteration (Prudhomme et al., 2003; Hirabayashi et al., 2013; Avand et al., 2021). Although warming may not trigger floods, it influences snowmelt and rainfall that can contribute to flood. Climate change can have positive as well as negative effects on pathogens and pests. It can lead to alterations in their population dynamics, abundance and diversity, biotypes, geographical distribution, interactions with plant, natural enemies, and extinction. Increased pests and pathogens infestation due to climate change are projected to cause reduced crop yields (Dhankher and Foyer, 2018).
3 Impacts of climate change on growth, uptake
of metals, and phytoremediation potential of plants
Phytoremediation potential (metal extraction and stabilization of contaminants) of plants depends on different factors like bioavailability of metal in soils, plant biomass, metal accumulation capability, microbiome, and climatic factors (Glick, 2010; Grčman et al., 2001; Miransari, 2011). Any abiotic parameter that influences these factors should also affect phytoremediation efficacy. Climate change and stresses associated with it such as elevated CO2 (EC), temperature, and drought can alter the plant growth and metal uptake and accumulation potential of plants. Different effects of EC, drought or warming, or a combination of climatic stress on metal accumulation and growth of plants differ greatly across plants used and chemical, biological, and physical characteristics of environment. These properties include pH of the soil, type of heavy metals and their bioavailable concentrations, diversity of microorganisms, and effects of climatic factors interaction (Rajkumar et al., 2013). Consequently, it is important to understand the interaction between climate change, growth of plant, and metal uptake and accumulation. Understanding the climate change effect on plant growth and metal accumulation can be beneficial when developing phytoremediation strategy for particular contaminated sites (Brunham and Bendell, 2011).
3.1 Impacts of elevated concentration of CO2
Elevated concentration of CO2 or EC alters the growth, metal uptake, and phytoremediation efficiency of plants (Table 1). It is widely recognized that EC can stimulate
Table 1 Effects of elevated CO2 on the heavy metal phytoremediation potential of plants.
Plant
Populus × euramericana (Dode) cv. “Nanlin-95” (NL95) one willow genotype (Salix jiangsuensis CL. “172” (J172))
Marvdasht cv. for wheat and Sepideh cv. for sorghum
800 + 50 μL L 1 0.06, 5.2, and 25.3 mg Cd kg 1
900 ± 50 μL L 1 0, 10, 20, and 40 mg Cd kg 1
Noccaea caerulescens 550±50 ppm
Cd 0.93±0.11 mg kg 1
Pb 91.2±5.6 mg kg 1
Cu 72.6±6.2 mg kg 1
Zn 188.2±11.8 mg kg 1
Increased Cd phytoremediation efficiency especially at high Cd concentration due to increased biomass
EC reduced the concentration of Cd in the roots and shoots of Cd-tolerant wheat, while it enhanced for Cdtolerant sorghum plants
Accumulation of metals and biomass
Guo et al. (2015)
Khanboluki et al. (2018)
Luo et al. (2019)
Sorghum vulgare × Sorghum vulgare var. sudanense hybrid and Trifolium pratense
Brassica juncea
Helianthus annuus
860 μL L 1 0, 300, 1500, and 3000 mg Cs kg 1
800 and 1200 μL L 1 100 and 200 mg Cu kg 1
Lemna minor
Robinia pseudoacacia
Robinia pseudoacacia
Lolium perenne and Lolium multiflorum
700 ppm 0, 1.5, 2.5, and 5 mg Cd L 1
700 ± 23 μmol mol 1 0, 1, and 5 mg kg dry soil 1
700±23 μmol mol 1 500.0 mg Pb kg dry weight soil 1
1000 ± 80 μL L 1 4, and 16 mg Cd L 1
Increased biomass and Cs accumulation
At EC (1200 μL L 1), both Indian mustard and sunflower showed increased biomass and Cu extraction capability
Wu et al. (2009)
Tang et al. (2003)
Cd concentration enhanced in plant Pietrini et al. (2016)
Three years of elevated CO2 decreased Cd uptake into leaves Jia et al. (2017)
3 years of elevated CO2 increased Pb concentration
Increased growth and Cd uptake
Jia et al. (2018)
Jia et al. (2011)
Arabidopsis thaliana 800 μmol mol 1 50, and 110 mM As Aboveground biomass and As concentration decreased Fernandez et al. (2018)
photosynthesis and plant biomass with the degree of stimulation being species or cultivar dependent (Ziska et al., 2012). Faster growth and greater biomass, in turn, could enhance phytoextraction and toxin uptake in aerial plant tissue. EC can augment plant biomass by 50% via CO2 fixation (Dier et al., 2018). It can enhance plant height, dry weight, and the leaf area by stimulating photosynthesis (Khanboluki et al., 2018). As CO2 increases, the stomatal pores don’t open as wide, leading to decreased conductance of stomata (gs), lower transpiration, and increased intrinsic water-use efficiency (WUEi) (Jarvis et al., 1999; Aranjuelo et al., 2006; Erice et al., 2006; Long et al., 2006). Högy et al. (2010) reported that EC (409 ppm versus 537) enhanced biomass and concentration of metabolites of low molecular mass in several weed species. In comparison with herbaceous species, heavy metal accumulator plants, poplars, and willows were found to be more EC responsive (Ainsworth and Long, 2005), as evident by their biomass, height, and carbon assimilation, which was suggested to be the result of stimulatory effect of EC on the photosynthetic rate and expansion of leaf (Curtis and Wang, 1998; Nowak et al., 2004). However, long-term continuous EC exposure may lead to a reduction (downregulation) of photosynthetic capacity. Often this decrease is accompanied by decreased photosynthetic enzymes and pigments such as ribulose‐1,5‐bisphosphate carboxylase/oxygenase (Rubisco) (Faria et al., 1996; Centritto and Jarvis, 1999). Goufo et al. (2014) observed that elevated CO2 increased the accumulation of carbon in rice throughout its whole life cycle.
ROS have been related to the inhibition of growth, utilization of nutrients, and photorespiration of plants. Since heavy metals increase ROS generation, it is hypothesized that CO2 can reduce damaging effects of metals on plants. EC levels led to increased plant growth, uptake of Cd, rate of assimilation CO2, efficiency of intrinsic water use and activities of antioxidative enzyme, decreased gs, transpiration rate and malondialdehyde (MDA), and no change in Cd concentrations in poplar genotype (NL95) and willow genotype (J172). It was proposed that EC promoted the growth of plant by boosting photosynthesis and increased phytoremediation efficiency, mainly at high Cd content (Guo et al., 2015). Cd accumulation due to EC differed in Cd-tolerant wheat and sorghum. EC reduced Cd contents in roots and shoots of Cd-tolerant wheat, while it enhanced for Cd-tolerant sorghum plants (Khanboluki et al., 2018).
Accumulation of metal and biomass in Noccaea caerulescens increased under EC (550±50 ppm) compared to controls (400 ppm CO2), suggesting that the EC could increase phytoremediation efficiency. Concentrations of water-soluble and exchangeable Cu and Pb decreased in soil under EC conditions, which decreased the risks of leaching of these metals. MDA concentrations in N. caerulescens reduced to varying degrees with the EC. It was hence suggested that EC can decrease oxidative damage resulting from metals in this species (Luo et al., 2019). EC (860 μL L 1) caused 32%–111% increased aerial biomass of the Sorghum and 8%–11% in Trifolium species in comparison with ambient CO2. It also caused up to 73% increased Cs accumulation in Sorghum and 43% in Trifolium species (Wu et al., 2009). At EC (1200 μL L 1), both Indian mustard and sunflower showed increased leaf area, biomass, and Cu extraction capability compared to when grown under ambient CO2 (Tang et al., 2003). Increased photosynthetic efficiency, Cd accumulation, antioxidant capacity, growth, and Cd phytoremediation capability of Lemna minor with increased Cd treatments, time of
exposure, and EC (350 and 700 ppm) were observed (Pietrini et al., 2016). Cd toxicity alleviation at low Cd treatments at EC in L. minor may be credited to enhanced photosynthesis and increased antioxidant capacity. Long-term EC increased the phytoextraction of Pb by Robinia pseudoacacia from contaminated soils (500 mg kg 1 soil) (Jia et al., 2018). Increased accumulation of Pb in leaves and removal of Pb from soils were observed. Width and height of seedlings increased due to EC in comparison with ambient CO2. EC led to increased SOD and CAT activities and higher concentrations of cystine, glutathione, phytochelatin, phenolics, proline, and flavonoids in plants under Pb treatment. EC (800 μL L 1) stimulated the growth of Sedum alfredii nonhyperaccumulating and hyperaccumulating ecotype but an increase was much greater in hyperaccumulating ecotype. EC was proposed to be an approach to increase phytoremediation efficiency of S. alfredii hyperaccumulating ecotype (Li et al., 2013).
EC increased Arabidopsis thaliana biomass (aerial) significantly. However, the level of stimulation of biomass was dependent on ecotype. At As concentration of 110 μM, the relative effect of EC was to reduce both As concentration and As uptake per plant; however, genetic variation was also obvious among A. thaliana for As phytoextraction at existing and expected CO2 levels (Fernandez et al., 2018). EC (360 and 1000 μL L 1) led to more increase in shoot biomass than in root biomass of Lolium perenne and Lolium multiflorum exposed to 4 and 16 mg Cd L 1 but reduced Cd concentrations in all plant tissues (Jia et al., 2011).
3.2 Impacts of elevated temperature
Temperature influences the growth and development rate of plants. It can also influence metal uptake by plants (Table 2). Each plant species has a particular temperature range characterized by an optimum, maximum, and minimum. Responses of plants to temperature increase depend on the plant development stage. For most species of plants, vegetative development generally has a greater optimum temperature compared to reproductive development. Temperature may affect water chemistry and growth of plants (Fritioff et al., 2005). It alters plant transpiration rate, metabolism, and growth and thus influences uptake and elimination of contaminants (Yu et al., 2005). Changes in temperature show the large impact on the uptake and accumulation of metals by plants. Therefore, it is imperative to understand the relation between temperature change, plant growth, and uptake and accumulation of metal when planning for phytoremediation in different bioclimatic zones (Brunham and Bendell, 2011). Fastest growth happens around 15 and 30°C in most plants (Larcher, 1995). Application of higher temperatures in the field can minimize the time duration required to attain clean-up aims. Baghour et al. (2001) observed higher Cr uptake by Spunta variety of Solanum tuberosum at high temperature compared to low temperature. Metal transfer from soil to plant was more at higher temperature in Potamogeton natans, Elodea canadensis (Fritioff et al., 2005), willow (Yu et al., 2010), and safflower (Pourghasemian et al., 2013) for various metals (Cd, Cu, Cr, Zn, Pb). This was suggested to be due to enhanced plant growth and uptake of metals by roots under warmer temperatures. Temperature increase (26 and 30°C) enhanced Cr toxicity by reducing photosynthesis and growth and increasing activities of antioxidative
Table 2 Effects of elevated temperature and water deficit on the heavy metal phytoremediation potential of plants.
Plant species
Elevated temperature/ water deficit
Atriplex halimus Elevated temperature 26 and 30°C 1 mM Cr
Oryza sativa Elevated temperature 40°C for 2 h
50 μM 100 and 500 μM Cd
Salix matsudana
Koidz × alba L.
Solanum tuberosum var. Spunta
Elevated temperature 11, 14, 17, 20, 22, 24, 26, 28, 30, and 32°C
16, 20, 23, 27, and 30°C
1.51 (± 0.01) and 1.53 (± 0.01) mg Cr L 1 for the treatment with Cr(VI) and Cr(III), respectively
Ag: 1 μg L 1
As: 2 μg L 1
Sb: 1 μg L 1
Cr: 2 μg L 1
Carthamus spp. 11–32°C 0, 0.5, 1, 5, 10, 20, 50, 100, and 500 μM Cd
Festuca arundinacea Water deficit
Soil moisture registered below 50% of control
Lolium multiflorum Soil was supplied with a fraction of water evapotranspired in wellwatered pots.
0.64 + 0.05 μg Se g 1
Reference
Enhanced Cr uptake and accumulation Mesnoua et al. (2018)
Increased Cd uptake and accumulation due to heat treatment in vacuoles, parenchyma, and vascular cylinder of root tissue
The removal rates of Cr(III) and Cr(VI) by plants showed a linear relation with temperature increase
Root zone temperature of 23–30°C most favorable for uptake and accumulation of Ag, As, Sb, and Cr
Enhanced relative uptake and concentration of Cd with increase in temperature
Increased Se concentration
Shah et al. (2013)
Yu et al. (2010)
Rural sewage sludge Cu
204.5 mg kg 1
Zn 730.5 mg kg 1
Ni < 25.0 mg kg 1
Mn 225.8 mg kg 1
Reduced concentration coefficient of Cu, Zn, Ni, and Mn in roots under drought conditions
Baghour et al. (2001)
Pourghasemian et al. (2013)
Tennant et al. (2000)
Pascual et al. (2004)
Triticum aestivum 70% of water-holding capacity 7.67 mg Cd kg 1
Beta vulgaris T1: applied 300 L/block each time, T2: applied 200 L/block each time, T3: applied 100 L/block each time; T1 was control
1.87 mg Cd kg 1
Brassica oxyrrhina 200 mg L 1 PEG Cu, Zn ranging from 300 to 1500 mg L 1
Drought treatment further reduced the biomass of plant and resulted in oxidative stress. In wheat tissue, drought increased Cd content Khan et al. (2019)
Shoot biomass and efficiency of Cd remediation potential also increased
Tang et al. (2019b)
Metal concentration decreased. Plant growth did not differ, greatly suggesting that drought stress did not make plants extra vulnerable to metal stress
Ma et al. (2016b)
Zea mays cv. single cross 704 Irrigated with different Cd concentration at 1-, 3-, and 7-day intervals
10 and 20 mg Cd L 1
Cd accumulation was more Azizian et al. (2013)
enzymes in Atriplex halimus. It also increased nutrient uptake and translocation but decreased Cr translocation. At 1 mM Cr treatment, Cr concentration in roots at 26°C was 7.2 g kg 1 and at 30°C was 9.1 g kg 1, respectively, whereas Cr concentration in the shoot was 0.45 at 26°C and 0.44 g kg 1 at 30°C (Mesnoua et al., 2018).
Shah et al. (2013) reported enhanced Cd uptake and accumulation in vacuoles, parenchyma, and vascular cylinder and stimulated antioxidant system in rice cv. Bh-1 roots subjected to a combination of heat stress and suggested that heat stress may mitigate mild Cd toxicity directly by an increased formation of Cd2 +-MT providing Cd2 + tolerance. The removal rates of Cr(VI) and Cr(III) by plants showed a linear relation with a temperature rise from 11°C to 32°C. The rate of Cr(III) and Cr(VI) removal was the highest at 32°C. Cr(VI) and Cr(III) translocation was observed from the root to the lower stem. Cr(VI) removal using hybrid willow was more vulnerable to alterations in temperature compared to Cr(III) (Yu et al., 2010).
Efficient transfer of metals from soil to plants is good for phytoremediation purpose. Therefore, improved understanding of how the temperature affects the metal bioavailability in cultivated soils is important. Dissolved organic matter (DOM) is the main metal complexing substance in soil, whereas soil organic matter (SOM) is the prominent metal-carrying phase in soil. Content and properties of the organic matter determine the metal complexation and mobility in soil. The temperature increase is suggested to stimulate the metal mobilization via influencing the SOM degradation and altering the metal complexation in water present in pores by changing the nature and quality of DOM (Cornu et al., 2011). In lettuce crop, a consistent decrease in bioavailability of Zn and Cd was reported from 10°C to 30°C, and this was credited to a strong metal complexation in the pore water because of a rise in soil temperature at higher temperatures. However, Zn and Cd transfer from soil to plant was stimulated at higher soil temperatures, suggesting that this process was affected most at higher temperature (Cornu et al., 2016).
In many cases, plant growing under high temperature condition showed the promotion of plant growth, increased photosynthetic rate, and accumulation of greater amount of heavy metals. Temperature-led indirect effects, such as SOM decomposition, on metal mobilization and/or uptake have been suggested the reason for higher metal accumulation (Sardans et al., 2008; Van Gestel, 2008; Conant et al., 2011).
3.3 Impacts of drought
Drought can be critical in areas with low availability of water or random alteration in weather during plant growth period. The effects of drought are likely to increase with climate change and growing water scarcity. It is a major environmental stress factor that affects the growth and development of plants. Understanding of relation of drought, plant growth, and metal uptake is necessary for phytoremediation under climate change. Variable effects of drought on phytoremediation potential of plants were reported in metal-polluted soils. In tall fescue, low soil moisture increased the accumulation of Se and reduced the production of biomass. Overall, drought reduces the heavy metal phytoremediation potential of plants (Tennant and Wu, 2000). High drought stress may harm many functions of plants but the main consequence is a
decrease of carbon fixation, which causes a decreased plant growth depending on duration of the stress, the stage, and the existence of other stresses. However, Angle et al. (2003) observed higher biomass and uptake of metal such as Zn or Ni by plants at higher soil moisture leading to greater concentration of metals extracted from soil in Alyssum murale, Berkheya coddii, and Thlaspi caerulescens Beta vulgaris plants grown in Cd-polluted soil [controlled deficit irrigation (300 L: T1, 200 L: T2, 100 L: T3)/block at each irrigation] throughout the organogenesis stage. The shoot biomass increased by 15.8%, and efficiency of Cd phytoremediation potential under T2 treatment (5.42 g ha 1) was 39.7% higher compared to T1 and 61.8% higher than T3 (Tang et al., 2019b).
Water is needed for many biochemical and physiological processes, including germination, division and elongation of cells, various metabolic activities including organic compounds synthesis, respiration, and photosynthesis (Lange et al., 2012). Drought leads to lower height, decreased leaf number and size, and less production of fruit. It also causes stomatal closure and increased resistance to CO2 diffusion in leaves (Alizadeh et al., 2015). Study on the structure of xylem and hydraulic conductivity revealed that metal stress exacerbates water stress in an additive manner and thus makes the Acer rubrum plants more sensitive to drought (de Silva et al., 2012). However, the effect of metal stress and combination of drought and metal toxicity on the plant growth did not differ greatly, suggesting that drought stress did not make plants extra vulnerable to metal stress (Ma et al., 2016b). Photosynthetic performance such as gs, chlorophyll fluorescence, and chlorophyll concentration declined. Water status of plant was the last affected parameter by the drought treatment (Ings et al., 2013). Water deficit also reduced the transfer and concentration coefficient of different metals, including Cu, Zn, Ni, and Mn in L. multiflorum Lam. roots in the soil in which rural sewage sludge was amended in high amount (Pascual et al., 2004). Drought reduced the transfer and concentration coefficient of some heavy metals in the root of sewage sludge (SS), mineral fertilizer (M)-treated plants, indicating that heavy metals in sewage sludge were preserved in chemical forms with low availability (Pascual et al., 2004).
Cd-treated wheat plants grown for 125 days after seed sowing had lesser biomass and greater oxidative stress and drought treatment further reduced the biomass of plant and resulted in oxidative stress. In wheat tissues, drought enhanced the Cd content (Khan et al., 2019). In Indian mustard plant, drought stress at 90 day after sowing led to a decreased Cd uptake (Bauddh and Singh, 2012). When corn plants were irrigated with water containing 0, 5, 10, and 20 mg Cd L 1 at 1-, 3-, and 7-day irrigation intervals, toxicity symptoms of Cd appeared clearly on the plant. Stem dry weight, transpiration, and height of plant were most sensitive to Cd levels. Cd accumulation was 24%, 56%, and 27% more at 1-, 3-, and 7-day irrigation interval in leaves compared to stems, indicating that corn can be useful for Cd phytoremediation under optimum water conditions and mild pollution of the soil (Azizian et al., 2013). As the success of phytoremediation technique relies on the plants’ capability of heavy metal accumulation and sufficient biomass yield, these studies revealed that drought might not exert significant positive effects on the phytoremediation of heavy metals.
4 Plant-microbe interaction and their effect on metal uptake and phytoremediation in a changing climate
Plant-associated microorganisms are an important factor influencing the metal uptake and growth response of plants to climate change (Compant et al., 2010) (Fig. 2). Exudates of plant roots are reported to increase the mobility of nutrients and heavy metals by inducing microbial activity indirectly and thus enhance the efficiency of phytoremediation (Ström et al., 2002; Pérez-Esteban et al., 2013; Sessitsch et al., 2013). Plant rhizosphere-associated microbial communities play a beneficial role in the growth of plants in metal-polluted soils (Xiong et al., 2010; Rajkumar et al., 2012). Many of these microbes boost the plant growth and uptake of nutrient by making nutrient available to plant through the solubilization of mineral nutrients like P, N, Fe, K, etc., production and excretion of plant growth-promoting compounds such as phytohormones, and release of specific enzymes (e.g., 1-aminocyclopropane-1-carboxylate deaminase). They also reduce metal led toxicity indirectly by stimulating defense mechanisms of plants. Microbes alter the bioavailability of heavy metals in soil by several mechanisms, including alteration of soil pH, metal translocation, chelation, complexation, acidification, precipitation, and redox reactions (Rajkumar et al., 2012; Guo et al., 2014; Ma et al., 2016a), and thus help in their uptake, sequestration, and detoxification from polluted sites. Different microbes adopt different mechanisms to activate either immobilization or mobilization and hence contribute directly to phytostabilization or phytoextraction (Ma et al., 2011). Some plant growth-promoting
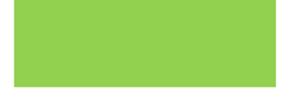
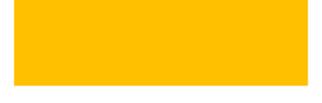
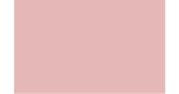
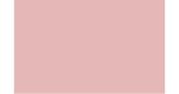
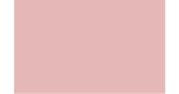
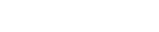
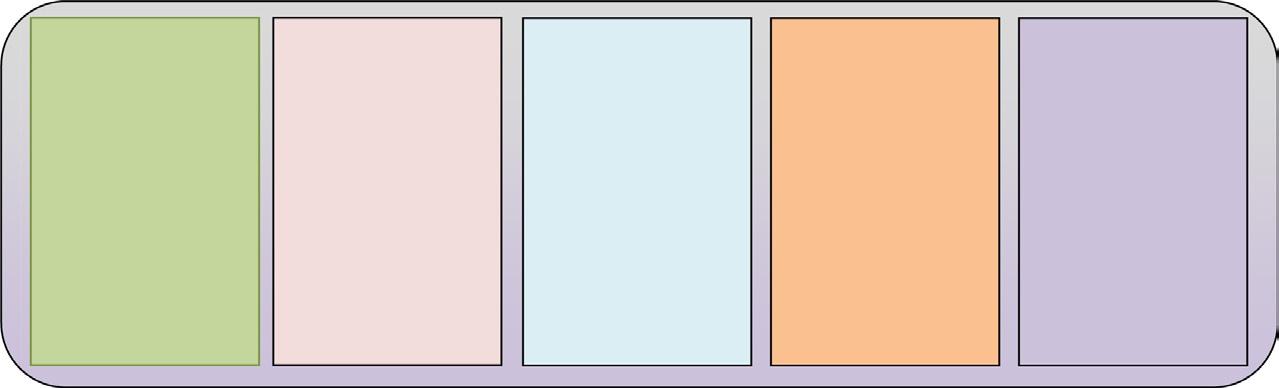
Fig. 2
bacteria (PGPB) produce siderophores, phytohormones, and biochelators; fix nitrogen; and solubilize mineral nutrients such as phosphate or potassium, which are beneficial for MAP (Ma et al., 2011; Rajkumar et al., 2012; Ahemad and Kibret, 2014). The impacts of adverse environmental conditions accompanying climate change, such like EC, drought, and warming, on beneficial plant-microorganism-metal interactions are increasingly being explored. Several studies have revealed the impact of climate change on plant-microbe interactions and how this affects phytoremediation.
4.1 Impact of elevated CO2
EC in general enhances the biomass production and metal accumulation in plants under climate changes. It generally helps plants to support greater microbial population and/or protects diverse microorganisms against heavy metal stress (Rajkumar et al., 2013). Generally, EC increases the growth and productivity of plant, resulting in an enhancement in input of carbon into soil, alteration in soil microenvironments including enhanced soil moisture, and promotion of activity and growth of microbes (Brett Runion et al., 2009; Lipson et al., 2005; Nelson et al., 2004).
In R. pseudoacacia seedlings, heavy metals led to decreased population and biomass of microbes in rhizosphere soils and altered microbial community too. Enhanced microbial populations, biomass, and changes in microbial community in R. pseudoacacia seedlings grown under EC and the presence of Pb, Cd, or Cd + Pb were reported. EC also enhanced the ratio of removal of Pb and Cd in rhizospheric soils. It can be concluded that EC can positively affect soil fertility, rhizospheric microenvironment, and heavy metal removal from soil (Huang et al., 2017).
In metal-rich soils also, EC leads to alteration in diversity and activity of microbes associated with plant roots. Generally, EC has a positive impact on abundance of ectomycorrhizal and arbuscular fungi but the effects on PGPB and endophytic fungi are variable. Root of Trifolium and Sorghum under EC in Cs-enriched soils consisted of large fungal and bacterial population but not actinomycetes compared to EC and Cs-contaminated soil. Microbial associations enhanced Cs accumulation by the plant without reducing growth (Wu et al., 2009). Song et al. (2012) observed increased actinomycetes, bacterial, and fungal populations and enhanced microbial N and C in the rhizospheric soils of Amaranthus cruentus and Phytolacca americana. EC increased biomass and triggered more Cs accumulation by plants. EC and inoculation of endophyte led to the stimulation of plant growth (enhancement in morphological properties of root and exudation, altered uptake, and distribution of Cd of both S. alfredii ecotypes) (Tang et al., 2019a). In rice rhizosphere, EC led to increased microbial respiration leading to reductive Fe(III) (hydr)oxide dissolution and oxidation of arsenite (Oremland and Stolz, 2003), as suggested by increases in Fe and arsenate concentrations (Muehe et al., 2019). Application of Burkholderia sp. D54 and EC enhanced the production of biomass and the accumulation of Cs in A. cruentus and P. americana than in control (Tang et al., 2011). Similarly, enhanced growth and phytoextraction efficiency were observed in rye grass grown in the presence of multiple heavy metal/metalloid (Zn, As, Pb, and Cd)-polluted soil (Guo et al., 2014). Perhaps EC reduces the negative effects exerted by heavy metal on rhizosphere-associated