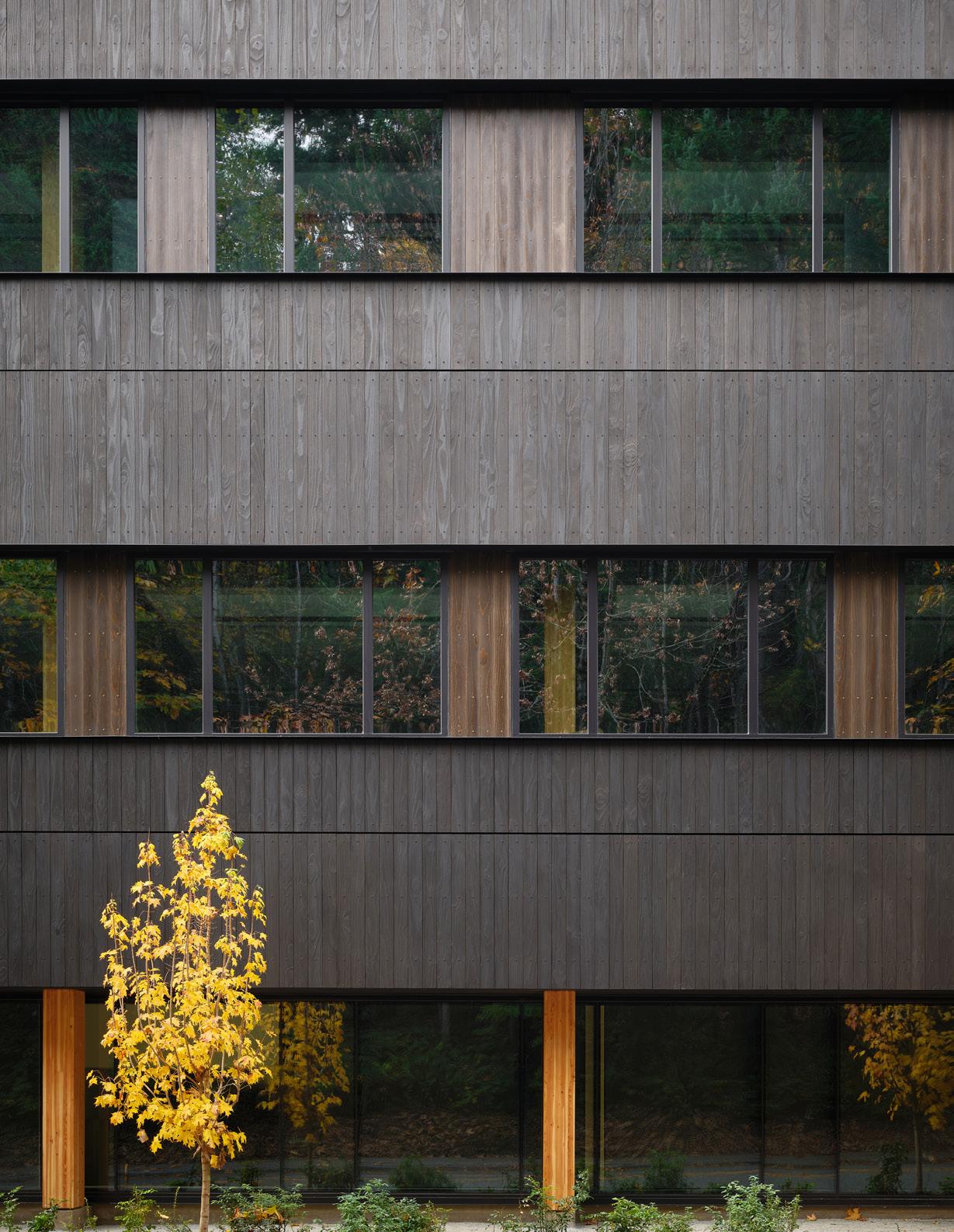
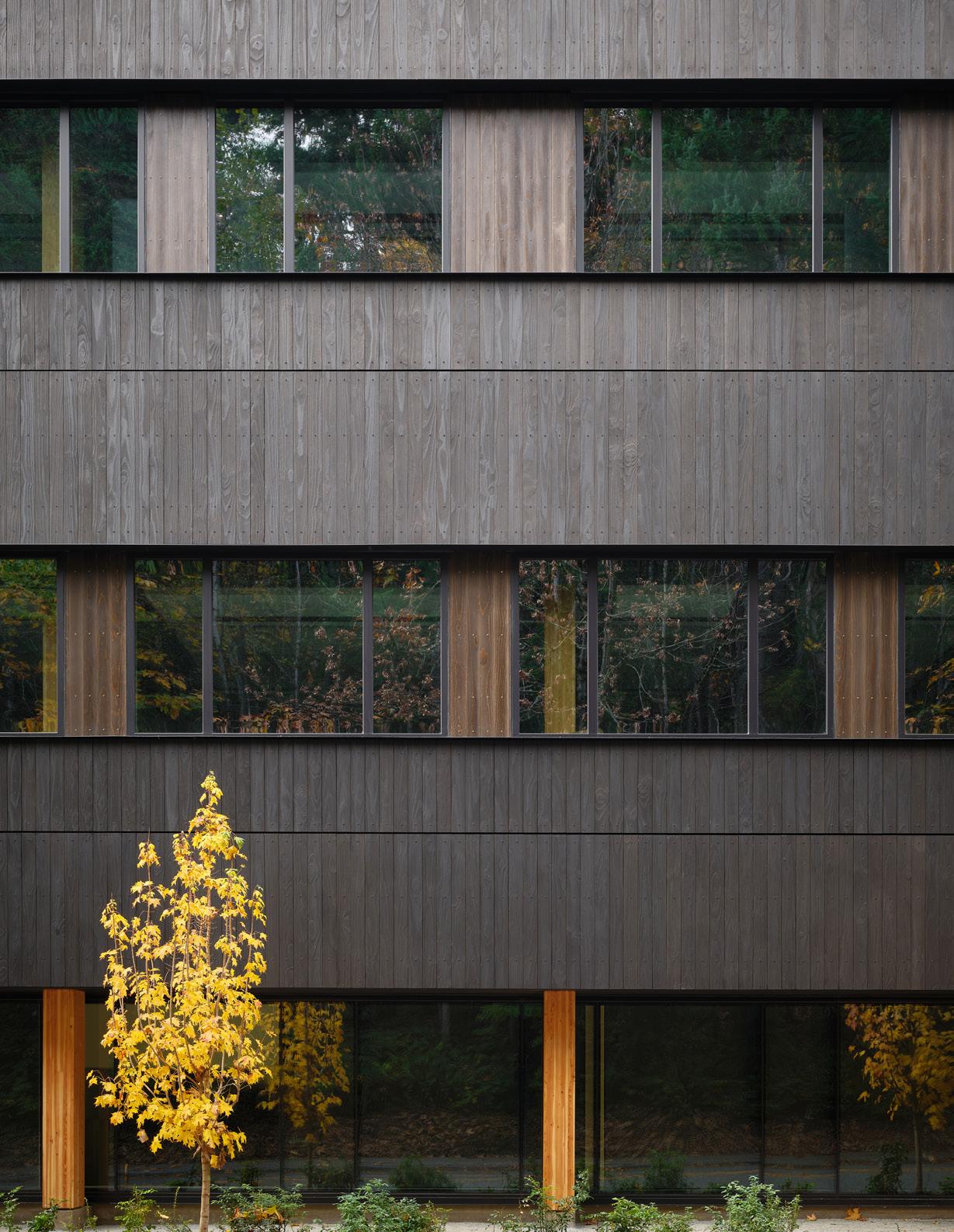
Embodied Carbon
Benchmarking Report
Acknowledgments
This study was made possible by the contribution of staff from across Perkins&Will. In addition to those named here, thank you to the Perkins&Will Living Design Leadership Council and to the many embodied carbon champions across the firm’s studios who are working to realize Perkins&Will’s firmwide commitment to decarbonization. We would like to acknowledge and thank the following for their expertise, dedication, and contributions to this effort:
Core Team and Project Management
Kendall Claus
Jesce Walz
Dalton Ho
Perry Ashenfelter
Project Director
Jason F. McLennan
Research Directors
Leigh Christy Yehia Madkour
Advisors
Kathy Wardle
Mary Dickinson
Aayushi Mody
John Nelson
Anna Beznogova
Editor
Joanna Jenkins
Life Cycle Assessment Modeling support was provided by:
Aaron Bergmann
Aayushi Mody
Adam Rosenthal
Adriana Portela
Alec Zebrowski
Ali Gholamabbas
Allen Suwardi
Alycia Schramm
Amy Brander
Ana Paredes
Anastasia Ciorici
Andrew Baltimore
Angel Suarez
Anna Beznogova
Anne-Philippe Kakou
Anthony DeEulio
Aparna Joijode
Avi Green
Bill Xu
Bomyeong Noh
Brandusa Bularca
Brittany McNairy
Carmen Trujilo
Casey Ozog
Chelsea Davis
Dalton Ho
Damian Ponton
Dan Qin
Daniel Kleeschulte
Daya Zhang
Elaine Zhang
Elham Soltani
Elizabeth Mikula
Eric Brossy de Dios
Eric Nebel
Evan Chakroff
Francesly Sierra
George Sassine
Hey-Young Lim
Hugo Santibanez
Isabella Moran
Janice Yeung
Jason Diamond
Jason Pieper
Jesce Walz
Jill Lin
John Stultz
Juan Yactayo
Julia Manion
Justin Kong
Kaaviyaa Nagarajan
Katherine Chin
Kathryn Watson
Kendall Claus
Kevin Elika
Ksenia Garrett
Laura Perez
Lauren Obermiller
Lincoln Linder
Louis Peiser
Lucas Simons
Luis Barajas
Madelyn Gulon
Manuela Londono
Mariel Dougoud
Mary Matos
Matt Seager
Max Moore
Max Sopher
Maxwell Rossner
Meghavi Patel
Melanie Baron
Mohamed Imam
Monet Hall
Nakisa Dehpanah
Nengi Njere
Nick Boyd
Nikki Liu
Patrick Cunningham
Perry Ashenfelter
Phil Tu
Phillip Keuhne
Preston Pape
Qin Lin
Rand Zalzala
Reza Takallouie
Ricardo Garcia
Ritzo Law
Robert Jackson
Ryan Kam
Selorm Kornyoh
Shanni Hannein
Sheryl Joy
Shhrruti Jain
Shiva Honarvar
Shreya Das
Skyler Johnson
Sonata Caric
Stephanie Tramutola
Steven Webster
Tad Neeser
Tom Gibbons
Tyler Hinckley
Vanessa Smith-Torres
Veronika Jonsson
Vivian Cheng
West Pierce
Yasmeen Barkat
Yingjing Ma
Yure Suarez
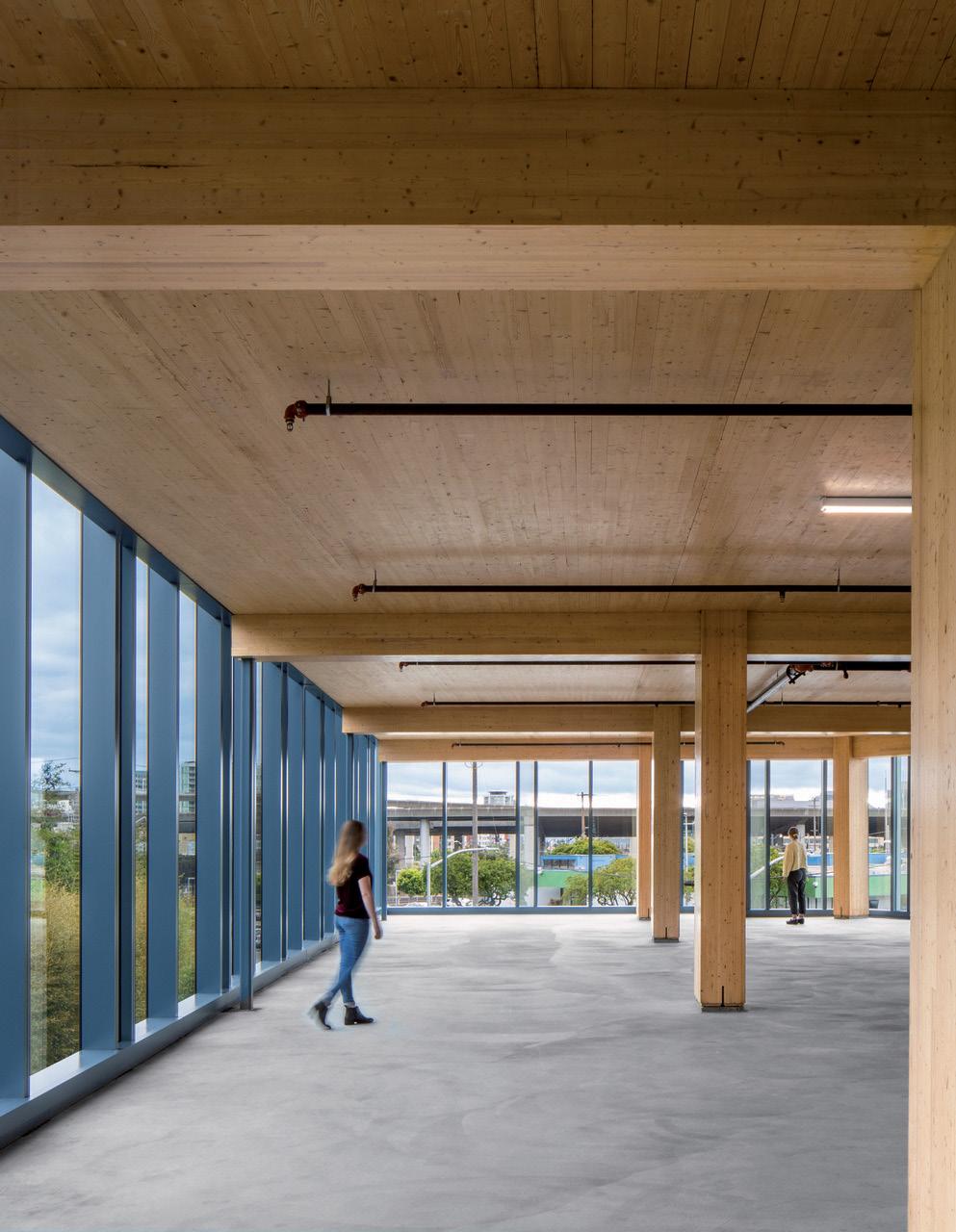
Executive Summary
Perkins&Will’s commitment to regenerative design, research, and decarbonization, coupled with its global presence, allows for the study of a large and diverse portfolio that spans across various scales, geographies, and building use types. This research was motivated by a desire to build upon the excellent body of existing studies and benchmarks, while continuing to contribute thought leadership to our shared decarbonization goals. Until recently, whole building embodied carbon benchmarks often relied on smaller sample sizes or data collected through inconsistent methodologies. This report aims to address these challenges.
We launched an ambitious firmwide effort to perform detailed embodied carbon assessments on a large sampling of our completed (or under construction) projects. This report is supported by a dataset of 89 buildings representing 14 building use types located across the United States and Canada, all designed by Perkins&Will, giving the researchers comprehensive access to project information. In parallel, our methodology was developed in alignment with the Carbon Leadership Forum’s embodied carbon “Benchmark Study V2,” to which we contributed both data and methodological input, reinforcing our commitment to industry-wide progress.
Our study included the following commercial building use types, aligned with the U.S. Energy Information Association Commercial Buildings Energy Consumption Survey (CBECS):
Laboratory (Academic)
Data Center
dataset represents projects that involve new construction, building reuse, core & shell, and varying scopes of interior fit out.
This research was motivated by the need to answer key questions sometimes left open to debate (or inadequately analyzed) by the embodied carbon information currently in the market:
a.
What building assemblies, components, or materials are the largest contributors to whole-building embodied carbon and how does it cascade from the largest to the smallest?
b.
How do building use type, geographic location, size, height, and other parameters affect a project’s embodied carbon? c.
What do these real-world findings uncover (or confirm), and what embodied carbon conservation measures are most effective in lowering a project’s overall embodied carbon impact?
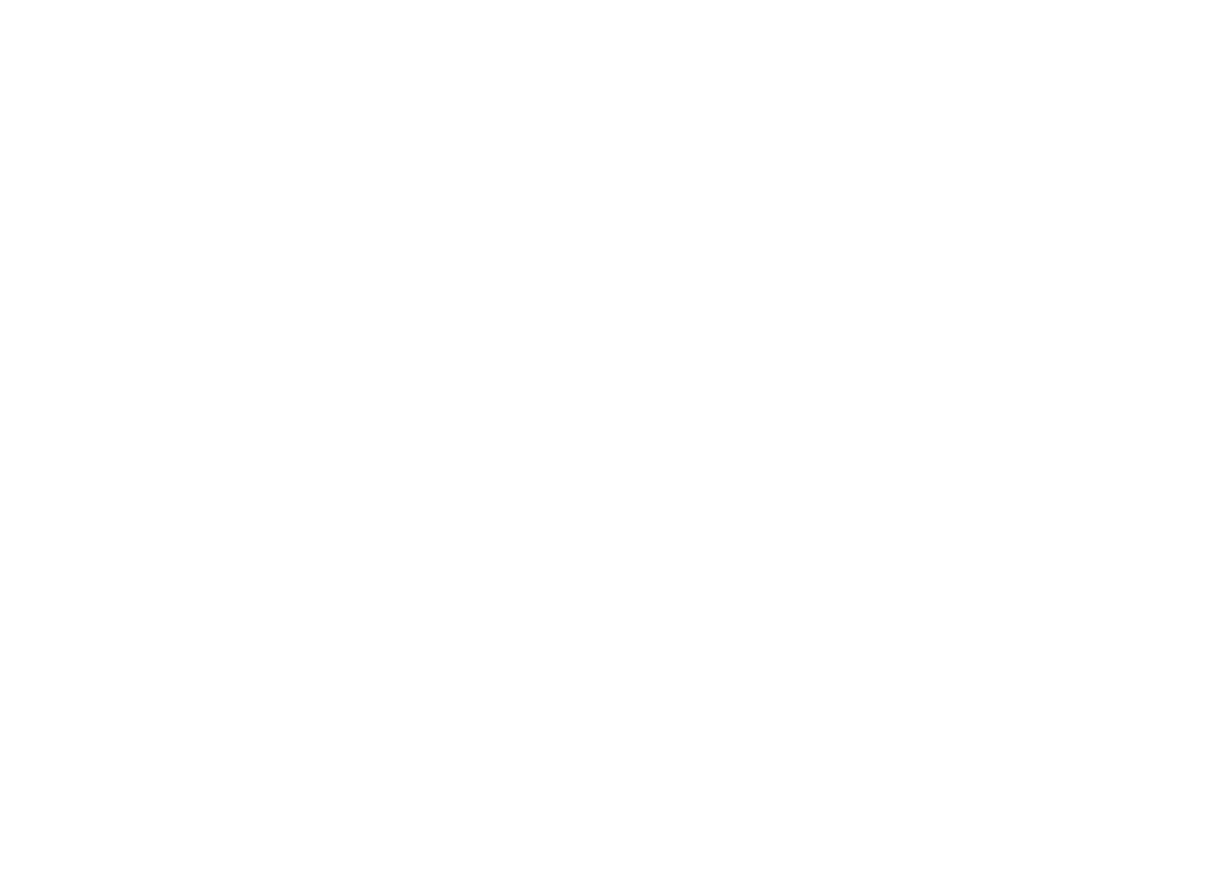
89 Studies
Following a standardized Whole Building LCA Methodology
18 Studios
Participation from a majority of our North American studios.
14 Building Use Types
To inform our Embodied Carbon Guidance
Figure 1: Location of all projects included in our Benchmarking Report.
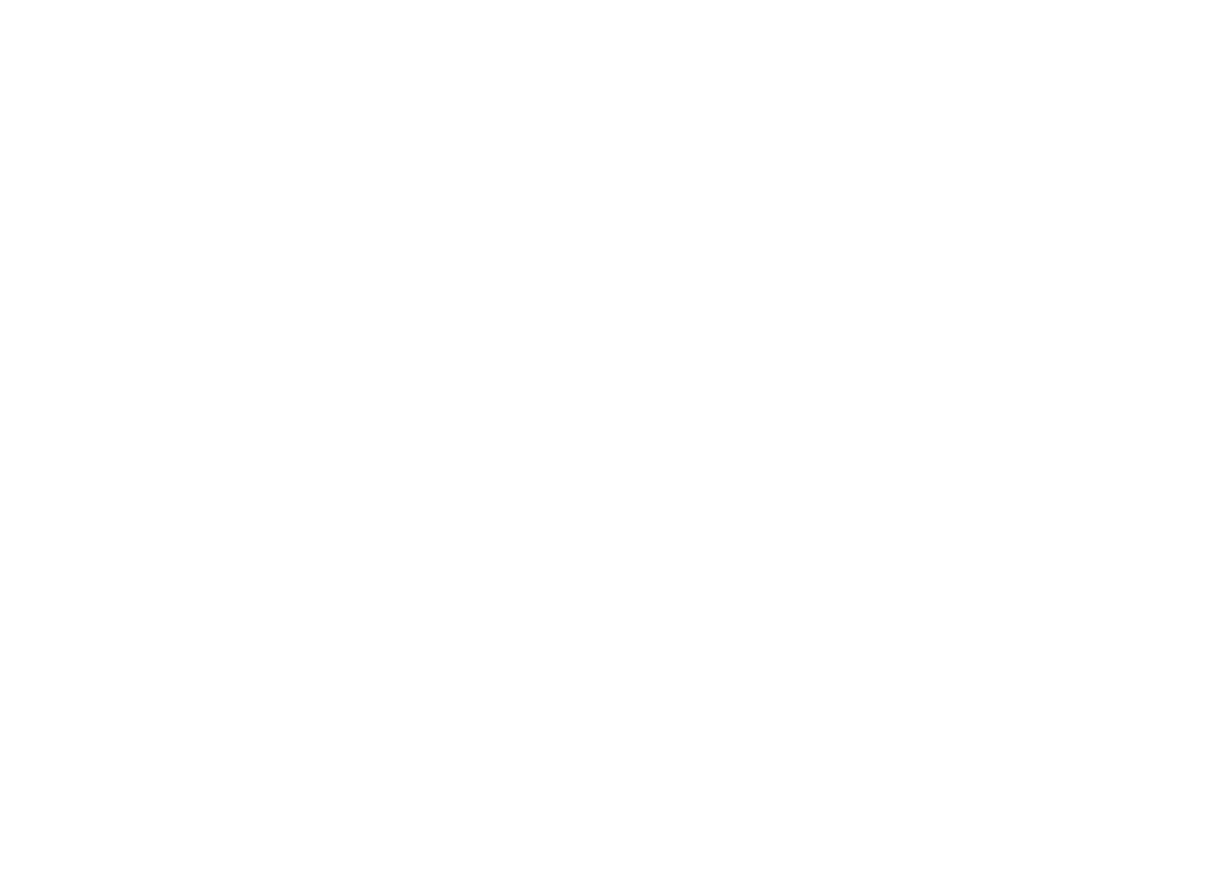
Embodied Carbon Impacts per Material Division
Whole Building LCA Benchmarking Projects by GWP intensity & material division (kg CO2eq / m2), Life Cycle Stages A1-A3, excluding biogenic carbon
The research conducted in this study revealed the following breakdown of embodied carbon contribution across primary material categories:
04 - Masonry, 2%
09 - Finishes, 4%
07 - Thermal and Moisture Protection, 6%
08 - Openings and Glazing, 7%
03 - Reinforcing, 10%
Figure 2: This figure shows material division's contribution to all benchmarked projects' embodied carbon intensity., or Global Warming Potential (GWP) per square meter (kg CO2e / m2). The data includes Life Cycle Stages A1-A3 and excludes biogenic carbon. See the "Methodology" section for more details.
03 - Concrete, 37% 06 - Wood/Plastics/Composites, 1% 05 - Metals, 33%
Figure 2 shows that concrete and metals are by far the largest contributors to a project’s embodied carbon. This remains consistent regardless of any distinguishing factor such as building use type, size, or location due to the inherently high global warming potential (GWP) of these materials, and the consistent need for every building to have concrete foundations and structural steel elements. This confirms what other studies have indicated about the proportional impacts of these materials; but this report begins to shed a more nuanced light on some key issues.
Since concrete and metals have such a large impact, they represent the first—and most meaningful— carbon reduction strategy design teams should address. It is also important to note that this includes concrete reinforcing (rebar), another large impact category, so careful sourcing of rebar goes handin-hand with a low carbon concrete approach.
Building Use Type
The percentage contribution of various construction materials varied slightly by building use type due to what was believed to be differences seen in the structural systems used to support these programs. While these differences aren’t believed to be large enough to be statistically significant, they do confirm the observation that buildings with more intensive programs, such as hospitals or laboratories, require more substantial superstructure and substructure compared to other building use types. This increases the proportion of the most carbon intensive materials (namely concrete and steel) verses aluminum, glass, or insulation used in building envelopes.
Building Enclosure and Climate
Geography has the potential to dictate the amount of material needed in the building’s structural system based on two key characteristics: climate zone (which introduces the need for more insulation for better thermal performance) and seismic zone (which affects structural requirements). Both have the potential to alter the material usage in the building, but for different reasons. In theory, cold climates warrant higher insulation values, while warmer climates require improved solar control. Similarly, projects in high seismic risk zones (or high wind areas) necessitate structural enhancements to accommodate these conditions.
› See the Findings section in the Introduction for further details on the relationship between structural systems, seismic and wind conditions, and embodied carbon.
Although a building’s insulation level (U-value) influences its embodied carbon, this study did not see appreciable differences across climate zones and building types in real-world projects. These results reiterate that added insulation to improve passive envelope performance should be prioritized over concerns about additional embodied carbon, especially when factoring in a building’s lifespan. However, it is important to note that various insulation materials do have appreciable embodied carbon differences when compared to each other, and the specification of insulation in particular must balance the sometimes competing needs of embodied carbon, thermal performance, and healthy materials.
Building Materials
Concrete and steel both exhibited the highest GWP regardless of structural system type and building use type. However, they both showed large variability within each area of study. This suggested that GWP intensities for concrete and steel systems are driven by more than just material choice alone: design decisions, code requirements, and reinforcement levels can significantly impact a project’s embodied carbon performance.
Since interior finishes tend to impact indoor air quality (due to the presence of potentially harmful chemicals in many conventional building materials installed in the breathing zone of regularly occupied spaces), the goal of using healthy interior materials should be carefully weighed against the goal of reducing embodied carbon. Selecting an interior material with a slightly lower GWP is not recommended if the trade off is the introduction of toxins or harmful materials that serve as respiratory irritants to occupants. This becomes especially acute in spaces where occupants may have compromised immune systems, such as healthcare spaces, or in K-12 schools as children’s immune systems are more sensitive than those of adults.
The lowest embodied carbon building is the one that already exists. This study reinforces what we already know inherently about the impact of building materials. The results show that adaptive reuse projects consistently demonstrate the lowest embodied carbon values across all building types. This holds true even when compared to mass timber structural systems, which still require new concrete and steel components. This underscores the value in reusing any portion of a project’s existing structural skeleton.
Operational and Whole Life Carbon
Lowering a building’s operational energy continues to be a primary factor of deep green projects as it allows for improved building resilience and less reliance on grid utilities. However, to understand the importance of embodied carbon as part of a building’s whole life (operational plus embodied) carbon footprint, this study compared the embodied carbon results with estimated operational carbon and found that embodied carbon makes up over half of a building’s whole life carbon emissions over the 60 year building life span of most building use types. This highlights the important need to address embodied carbon for any building type—even for all-electric and low-energy buildings where embodied carbon will make up the majority of whole life carbon emissions since other emissions have been reduced.
The objective of this report is to summarize the extensive embodied carbon research performed by Perkins&Will, thereby enabling informed decision making in the design and construction industry. The following sections outline detailed information on the projects in the study, the principal findings, and the methodology used in the research.
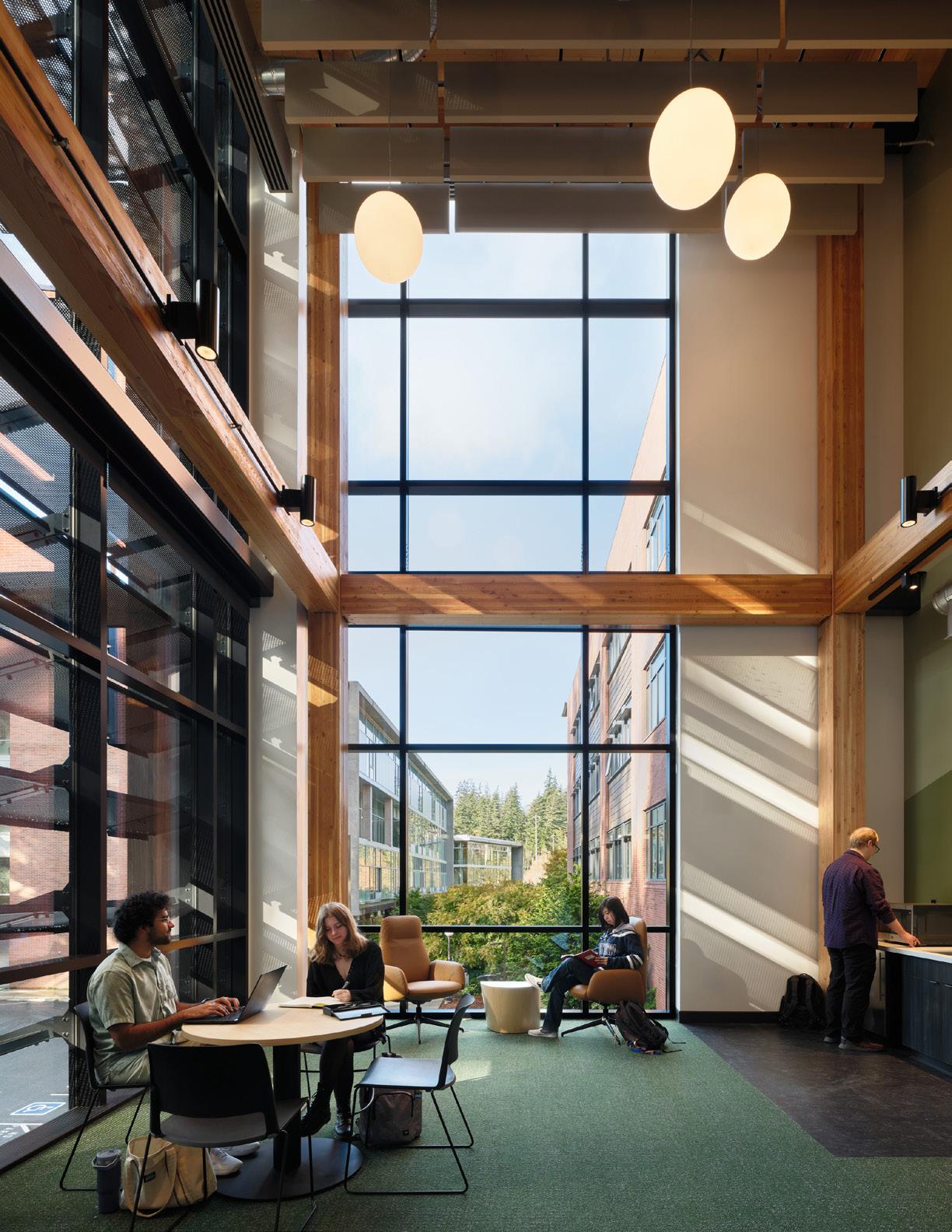
Introduction
Background
Embodied carbon refers to the global warming potential (GWP) associated with materials and services throughout their life cycles, which span from raw material supply through manufacturing, transportation, installation, use, and end of life. The embodied carbon impacts from building materials are a significant contributor to global climate change, making low carbon design and construction a vital ingredient in Perkins&Will’s mission towards a thriving and regenerative future. To put things into perspective, global cement manufacturing in 2022 equated to approximately 8% of global carbon dioxide (CO2) emissions. If the cement industry was a country, it would be the third or fourth largest emitter of CO2 in the world (source: World Economic Forum). Life Cycle Assessment (LCA) is a systematic method for calculating the environmental impacts of materials, products, and services throughout their life cycles. Whole Building LCA (WBLCA) calculates these impacts for a building over a reference study period (e.g., 60 years). LCA results provide data on GWP, as well as other impacts such as ozone depletion, eutrophication, acidification, and depletion on non-renewable resources. This study focused on GWP.
Operational carbon refers to the carbon emissions generated by the energy used to operate a building. Together with embodied carbon, the two terms represent the whole life carbon (WLC).
Unlike operational carbon—which comes from energy use during the building’s occupancy—upfront embodied carbon occurs before a project opens its doors, making early design decisions critical to reducing these impacts. Figure 3 shows the potential to reduce the carbon impacts of a project over its lifespan. Best practices for carbon reduction begin with reuse and optimization and continue through material selection and planning for adaptation and future reuse. While some projects may offset their impacts to achieve “net zero” goals, this should always be a last step after all other means of reduction have been implemented.
1
Figure 3 is a graphic representation of the relative impact that specific decisions can have on the project over time. GWP is quantified in kilograms of CO₂ equivalent (kg CO₂e).
“Equivalent” in CO₂e means that many greenhouse gases, including methane, nitrous oxide, and hydro fluorocarbons, are normalized to an equivalent amount of carbon dioxide based on their respective GWP and combined in a single metric.
The life cycle of buildings comprises four main stages:
1. A1-A5 (Product and Construction/ Installation Stage)
2. B1-B5 (Use Stage)
3. C1-C4 (End of Life Stage)
4. D (Loads and benefits that occur after the building’s usable life span)
Figure 3: Potential for impact reduction, per the World GBC Net Zero Carbon Buildings Commitment. Adapted from HM Treasury & World GBC 1
LIFE CYCLE STAGES
Stages not
Some of the WBLCAs performed in this study included all life cycle stages, but the results and findings are drawn from the impacts from life cycle stages A1-A3. This study focuses on A1-A3 for three reasons:
1. Approximately 80% of the embodied carbon from this analysis can be traced to the A1-A3 life cycle stages, also known as the “Product Stage.” This is in part because this study focused primarily on structural and envelope materials, which are not replaced as frequently as interior elements.
2. Impacts from Stages B, C, and D are less well documented and measured compared to the data used in A1-A3. While it is crucial to plan for building use, adaptation, reuse, and eventual end of life, the design decisions with the most immediate impact pertain to early design, material selection, and product specification, which are captured in A1-A3.
3. A4 and A5 impacts have been steadily gaining attention, and they rely strongly on participation from the project’s general contractor. Reliable access to this data was not available for most projects in this study.
Cradle to Grave
Cradle to Gate
B6 - Energy Use
captured in LCAs
Additional stages captured in LCAs Stages used in report
B7 - Water Use
Figure 4: Life Cycle Stages of an LCA as defined by EN 15978
This report includes findings from analyzing over 89 Perkins&Will projects of varying use, scale, construction type, and geographical location. The projects chosen to be included in this study represent real buildings that are modeled to a construction documentation level of detail. The buildings represent 14 use types, including office, education, lodging, entertainment/ assembly, laboratory, healthcare, order & safety, and data center.
LCA Benchmarking - Building Use Types
study per primary building use type (count)
WHOLE BUILDING LCA BENCHMARKING - BUILDING USE
Number of
(Higher-Education)
(Commercial)
(Academic) Entertainment (Civic / Assembly)
Healthcare (Inpatient)
Lodging (Multi-family)
Education (K-12)
Entertainment / Assembly (Rec / Community Center)
Order & Safety
Lodging / Residential (Hotel or Student Housing)
Entertainment / Assembly (Arena or Stadium)
(Outpatient)
Figure 5: Whole Building LCA
The individual use types can be broken down even further into the following:
VS. REUSE PROJECTS
PROJECTS WITH INTERIORS
Seventy of these projects were new construction, 19 considered “reuse” or “transformation,” and 68 included interiors.
The physical scope of this study included sub-structure, superstructure, and enclosure, with interior components assessed only if they were modeled. Since interior scope varied across projects, some included only core & shell (C+S), while others had full interior fit outs. In cases where interiors were included, the study accounted for finishes (ceilings, walls, flooring) and partitions (framing, insulation, and fenestration), but casework, furnishings, and MEP systems were excluded.
Figure 5B: Number of New vs Reuse Projects
Figure 5A: Number of Projects including Interiors Scope
Project Dataset Summary
The projects in this study ranged in size from less than 1,000m2 (10,760 sf) to more than 80,000 m2 (861,00 sf). Stories above grade ranged from one to over 20 stories, with a few outlying high-rises at close to 40 stories tall. However, the majority of projects fell between the 20,000 m2 to 60,000 m2 range (around 215,000 sf to 650,000 sf) and 2-10 stories. This wide spectrum of project sizes allowed for robust and comprehensive data from which to derive findings. See Figure 6A. Figure 6B shows the number of stories in our dataset.
Figure 6A: Gross Floor Area and Count of Projects per GFA Group (GFA in m2, including attached parking)
Figure 6B: Stories Above Grade
8 (Subarctic/Arctic)
7 (Very Cold)
6B (Cold Dry)
6A (Cold Humid)
5B (Cool Dry)
5A (Cool Humid)
4C (Mixed Marine)
4A (Mixed Humid)
3C (Warm Marine)
3B (Warm Dry)
3A (Warm Humid)
2B (Hot Dry)
2A (Hot Humid)
1A (Very Hot Humid)
Climate Zone
As shown in Figure 7B, most of the projects (23) in the study are located within Climate Zone 4C, Mixed Marine, which covers coastal Washington and British Columbia, Canada. This is followed by 13 projects in Climate Zone 4A, Mixed Humid, and 12 projects in Climate Zone 5A, Cold Humid. None of the studied projects were located within Climate Zones 4B or 5C.
Figure 7B: North America Climate Zone Map. Source: ASHRAE 169 - 2013
Figure 7A: Number of Projects per Climate Zone
Structural Systems
The projects in the dataset include a variety of primary structural systems. Figure 8A shows the breakdown of primary structural systems; most of the projects (31) use structural steel, and the second largest group use reinforced concrete (23). Eleven of the projects reuse existing structures, and the remaining projects use hybrid or mass timber structures.
# of projects per Primary Structural System
Figure 8B shows the breakdown of foundation types employed by the projects in the dataset. The majority (52) of the projects have a shallow foundation, 27 have a deep foundation (less than 50 feet), six have a deep foundation (greater than 50 feet), and four have some other foundation system.
of projects per Foundation Type
Reuse / Transformation
Hybrid (Concrete and Steel)
Concrete and Masonry
Hybrid (Timber with Concrete and/or Steel)
Mass Timber
Reuse with Timber Structure
Reuse / Transformation
Hybrid (Concrete and Steel)
Concrete and Masonry
Hybrid (Timber with Concrete and/or Steel)
Deep Foundation < 50 (15m) Deep Foundation > 50 (15m) Other Foundation System
Figure 8A: Number of Projects per Structural System
Figure 8B: Number of Projects per Foundation Type
Design
Figure 9 summarizes the seismic design category of the projects and their respective International Building Code (IBC) construction types. Most of the projects (28) are located in seismic design category D. This design category is rare for most of the U.S. but highly prevalent on the West Coast, and it requires buildings to meet more stringent structural design requirements. As for IBC Construction Type, Type I-A and I-B buildings (typically steel, concrete, or masonry structures with fire protective assemblies) were the most prevalent.
Figure 9: Number of projects per IBC Construction Type
Summary of Findings
This work primarily explores the relationship between building use type, structural system types, and embodied carbon, however, the findings also address the impacts from building location, height, and area.
Note: “n” denotes sample size.
Building Use Types
Building Use Types and Embodied Carbon Intensity (ECI): The findings indicate that building use types do not appear to be a strong factor dictating a building’s ECI. All building use types studied, except for order & safety (n=3), resulted in mean and median ECI’s within 250-450 kgCO2e/m2. However, the range of low to high values within each building use types can vary significantly, suggesting drivers outside of use type may dictate overall ECI.
› See Figure 13.
Building Use Types with High ECIs: The findings show that order & safety buildings demonstrated the highest ECI values (over 700 kgCO2e/m2). We associate this with the unique design conditions and requirements of these building use types, such as the need for higher carbon intensive materials to support building functionality.
› See Figure 13.
Building Use Types and ECI Range: Most building use types were found to be within a +/-200 kgCO2e/ m2 spread between high and low ECI results. For order & safety, higher education, and assembly use types we saw a higher spread (+/-300 kgCO2e/m2).
Several office buildings (n=24) demonstrated some of the lowest ECI results, below 300 kgCO2e/ m2, as well as a wider ECI range or variability. We associate this with greater design flexibility within the structure and enclosure from high-performing mass timber offices to traditional concrete building development. The office building use type also included more case studies than others, which may have contributed to the wider spread.
› See Figure 13.
Underground Parking vs Above Grade (within building footprint) Parking: In our findings, belowgrade parking (300 kgCO2e/m2) (n=10) increased structural ECI by roughly 30-50% compared to above grade (200 kgCO2e/m2) (n=5). It’s important to note that the below grade parking showed a much larger range (200-500 kgCO2e/m2), while the above grade parking only showed a range of 150-250 kgCO2e.
Structural System Impacts
Total Structural Impacts: This study confirmed that a building’s structure (superstructure and substructure) consistently made up the highest GWP proportionally across most building use types and on average made up 60-80% of building’s total GWP.
› See Figure 10
Superstructure: Superstructures (columns, beams, slabs, upper floors) typically accounted for about 50–60% of total GWP, while substructures (foundations, below-grade structure) accounted for 10–20% of total GWP.
› See Figure 12
Concrete and Steel Structures (Combined Super and Substructure): This study found that the mean for concrete structures and steel structures had the same mean ECI between 250-275 kgCO2e/m2. Both systems demonstrated a similar +/- 300 kgCO2e/ m2 range from high and low results. It’s important to note that the methodology used in our study removed product-level variations for concrete. Including product-specific GWPs will likely widen the range of concrete structural system impacts.
› See Figure 14A
Concrete and Steel Superstructure-Only: Our findings for concrete and steel superstructures also showed similar mean values between 200 - 225 kgCO2e/m2. In this analysis, steel superstructures showed a wider range from high to low results, indicating large variation in how steel structures are designed.
› See Figure 14B
Mass Timber versus Concrete or Steel: In this study, mass timber structural systems were on average over 30% lower than comparable concrete or steel structures before accounting for biogenic carbon. This is expected as mass timber does not require an energy intensive manufacturing process and is significantly lighter than steel or concrete. It is important to note that procurement is a strong consideration for mass timber. Ecological or biodiversity impacts from the planting, harvest, and transportation of the raw timber is not captured in the carbon assessment and can outweigh the embodied carbon savings if not procured responsibly.
› See Figure 14A.
Seismic Design Category and Seismic Site Class:
Seismic design category did not appear to show a strong correlation to ECI. Categories A through D all resulted in mean and median values between 200–250 kgCO2e/m2 and the same was found for site class that had varying mean and median values.
Climate Zone and Embodied Carbon: Despite a dataset of projects covering nine different climate zones, the study did not find a correlation between climate zone and embodied carbon. This is likely due to the wide variety of enclosure designs and thermal performance requirements. Also, structure and sub-structure impacts dominate the ECI values such that variations in envelope performance are less likely to show up. As noted above, this reinforces the recommendation that projects should not sacrifice thermal envelope performance for embodied carbon savings.
› See Figure 16
Building Geography, Height and Area
Building Area and GWP: This study did not find a strong connection between building area and GWP. It’s important to note that the dataset consisted primarily of projects smaller than 50,000 m2 (538,200 sf).
› See Figure 18
Stories Above Grade and Structural GWP: This study did not find a strong connection between stories above grade and structural GWP.
› See Figure 17.
The dataset consisted primarily of buildings between 2-10 stories (n=63), and the findings showed that mean and median values fell between 300-350 kgCO2e/m2, a relatively tight range. The study found that low-rise (1 to 5 stories) buildings had a wider ECI range, between 150–550 kgCO2e/ m2. The result shows that design decisions tend to have a stronger impact on ECI in low rise buildings (compared to taller buildings).
› See Figure 17
Envelope and enclosure made up 20-40% of a building’s ECI: After a building’s structural system, its enclosure makes up the next largest contributor. The contribution of enclosure to overall GWP varies greatly and is tied closely to material selection. The main contributors for envelope are metals, glass, conventional (high GWP) XPS insulation, and high GWP cladding.
Foam insulation: Conventional extruded polystyrene rigid foam (XPS) and closed cell spray foam with high GWP blowing agents (from hydrofluorocarbons, or HFCs) have disproportionately high carbon emissions due to their manufacturing processes and off-gassing after installation. This study confirmed that conventional XPS is a top contributor to GWP when used.
Window-to-wall ratio and GWP: This study did not find a strong link between window-to-wall ratio (WWR) and ECI. However, this study confirms that metals and glass are high contributors to building envelope ECI. This suggests that glazing and storefront should be balanced carefully between daylight harvesting, passive energy savings, and reducing WWR to improve overall thermal envelope performance.
Envelope and Embodied Carbon: With the selection of the right insulation material, improvements to passive envelope performance over the building’s lifespan should be prioritized over the additional embodied carbon from added insulation. However, we recognize that there is a point of diminishing returns and recommend that the optimal insulation levels should be determined by a parametric energy model.
Climate Zone and Embodied Carbon: Despite a dataset of projects covering nine different climate zones, the study did not find a correlation between climate zone and embodied carbon. This is likely due to the wide variety of enclosure designs and thermal performance requirements as well as varying building use types across these zones. Also, structure and sub-structure impacts dominate the ECI values such that variations in envelope performance are less likely to show up. As noted above, this reinforces the recommendation that projects should not sacrifice thermal envelope performance for embodied carbon savings.
Material Contributions and Savings
Concrete, metals (including steel rebar and structural steel), and insulation consistently showed higher GWP compared to other material categories. The use of concrete and steel can’t be entirely avoided in construction, however optimization options are available.
› See ECCMs in the Conclusion
Concrete is the single largest material contributor to embodied carbon in most buildings. It ranked as the highest GWP-intensity material in 10 out of the 14 building use types analyzed. This is due to both the large quantities of concrete used in structures and its high carbon intensity (from cement production).
Metals (steel rebar and structural steel) are the second-largest contributor to embodied carbon in most cases. Steel elements (reinforcing in concrete and steel framing members) have a high unit carbon intensity and are used extensively in structures, making them the next biggest factor after concrete.
XPS insulation: Conventional XPS insulation can be a large contributor to buildings’ GWP. This is particularly true in situations where conventional XPS is used for below-slab or roof insulation. This material is now banned in several U.S. states and in Canada; however, it is still common in regions where it remains legal to use. However, low-GWP XPS is now readily available, and its impacts are 90% lower than conventional XPS. Low or no-HFC insulation should be specified wherever XPS insulation or closed-cell spray foam are required.
Targeted low-carbon procurement can yield 10–50% reductions in material emissions. Choosing specific lower-carbon products within these highimpact material categories can dramatically cut embodied carbon. For instance, using an optimized concrete mix or higher recycled content steel, or swapping to a lower-GWP insulation, showed potential to reduce that material’s emissions by anywhere from 10% up to 50% in various projects.
Impact of Renovation and Adaptive Reuse
The greenest building is the one you already have. This study shows that adaptive reuse projects consistently resulted in the lowest ECI across all building use types. This holds true even when compared to mass timber structural systems which still require new concrete and steel elements. This underscores that the greenest building is often the one you already have and reusing any portion of the existing structural “skeleton” is the best strategy for immediate carbon reduction.
Savings from adaptive reuse generally relate to the percentage of floor area with an existing structure that is retained. Retaining 50% of a building’s floor area and associated structure generally saves 30-40% of total GWP as the structure generally makes up 60-80% of total GWP. On average, adaptive reuse projects in this study saved about 39% of total GWP when compared to equivalent new construction. Some reuse projects went even further, achieving up to 80% less embodied carbon than building new. These savings come from retaining the existing envelope as well.
Operational and Whole-Life Carbon
The whole life carbon of a project comprises both operational and embodied carbon. The carbon profile of a highly energy efficient building (low operational carbon building) has a proportionally larger contribution from embodied carbon. All building types, whether they have inherently high or low energy demand end uses, should address both operational and embodied carbon to lower whole life carbon.
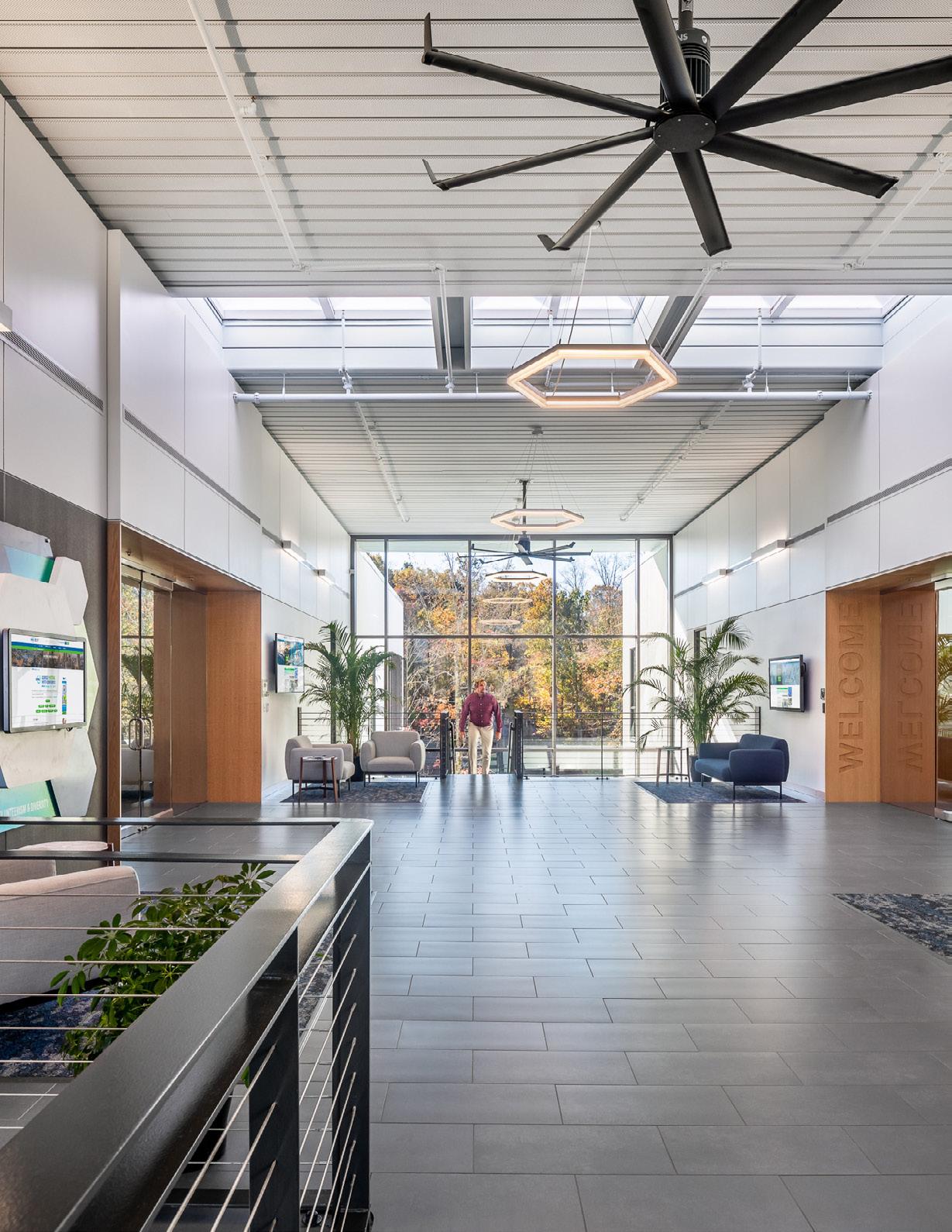
How to read Box Plot Diagrams
A box plot (aka box and whisker plot) uses boxes and lines to depict the distributions of one or more groups of numeric data. Box limits indicate the range of the central 50% of the data, with a central line marking the median value. Lines extend from each box to capture the range of the remaining data, with dots placed past the line edges to indicate outliers.
Results and Findings
This chapter organizes the results into two sections:
1. The Building Use Types and Structural Systems section addresses whole building results and shows analysis for discreet building assemblies independently from one another. This section is subdivided as follows:
a. Whole Building Foundation, superstructure, enclosure, and supporting elements such as insulation, doors, windows and primary interior assemblies
b. Building Structure and Enclosure Foundation, superstructure, enclosure only (columns, beams, slabs, and upper floors)
c. Superstructure
Primary structural assemblies only
d. Substructure
Source: A
Foundation and below-grade structure only
This section focuses on building structural systems and building materials, helping to pinpoint which components and assemblies are the largest contributors to GWP.
2. The Building Geography, Height, and Area section addresses the impact of climate zone and design parameters such as number of stories, area, and adaptive reuse. This section focuses on understanding how design decisions impact embodied carbon.
Complete Guide to Box Plots | Atlassian
Building Use Types and Structural Systems
Whole Building Analysis
The ratio of GWP intensity per material division varies across building use types, reflecting differences in structural, enclosure, interior, and other material demands. Superstructure and substructure consistently contribute the highest GWP across most building use types, with superstructure generally accounting for 50%-60% of impacts. Notably, order & safety buildings showed higher superstructure impacts (around 70%), while outpatient healthcare buildings exhibited lower impacts (around 35%). Impacts from substructure ranged from 10%-20% across many building use types except for stadiums and arenas, which showed a distinct distribution with a significant share attributed to “other” materials.
Certain building types—such as Education (K–12), outpatient healthcare, lodging (hotel/student housing), and data centers—had higher enclosure impacts compared to others, around 30%. The interior scope, which was not consistently included across projects, showed a wide range of impacts (2%–22%). Lower contributions were observed for stadiums and arenas, data centers, and order & safety buildings, while higher contributions were noted for healthcare buildings, higher-education buildings, and multi-family buildings.
BUILDING ELEMENT
While building use type might not be the primary determinant of a building’s embodied carbon footprint, Figure 10 reveals noteworthy patterns that could inform future projects. These findings underscore the importance of tailoring embodied carbon reduction strategies to the specific demands of each building use type.
REVIT BUILDING ELEMENT
Figure 10: Projects by GWP Intensity and Material Division
The rankings in Figure 11 show that concrete and steel represent the most GWP intense materials, regardless of building type. Concrete ranked as the highest intensity material in 10 out of 14 building use types, and steel was the second highest contributor in the remaining three building use types. Insulation, cladding, and aluminum were also strong contributors to GWP, although with varying impacts across building use types.
When accounting for whole building scope, the mean GWP intensity across building types spanned from approximately 250-450 kg CO₂e/m², with notable exceptions. Many types, including healthcare (both inpatient and outpatient), lodging (both hotel/student housing and multifamily), office buildings, and data centers, exhibited relatively similar median GWP intensities within a moderate range of 300–400 kg CO₂e/m². This suggests that building use type alone doesn’t necessarily drive embodied carbon intensity.
Public Assembly (Civic)
Assembly (Rec./Community)
Assembly (Stadium/Arena)
Education (Higher Education)
Education (K-12)
Healthcare (Inpatient)
Lodging/Residential (Hotel/Student)
Lodging/Residential (Multi-family)
Office
Other (Data Center)
Other (Order & Safety)
The set of graphs in Figure 12 illustrate the GWP intensity breakdown for new construction projects. The sunburst charts highlight that superstructure components, particularly floors and structural framing, contributed the largest share of total GWP (around 55%), followed by enclosure (19%), and substructure elements (15%). The bar charts reinforce this idea and show that concrete, metals, and insulation are the dominant contributors to overall GWP, especially for floors, walls, structural framing, and foundations. Cast-in-place concrete led in material-specific impacts, while steel, mullions, and aluminum also represented significant GWP sources. It is important to note however, that increased insulation does not make a large enough difference to overall GWP to justify a reduction in thermal per formance.
Wood/Plastic/C...
Structural
Structural
Structural
Curtainwall
Curtainwall
Figure 12: Contribution Analysis Across All New Construction Projects (metric tons CO2eq),
REVIT BUILDING ELEMENT
Structure and Enclosure Analysis
In Figure 13, our study compared building use types but limited scope to isolate structural and enclosure system efficiencies. All building use types except for order & safety, resulted in mean ECI’s between 250-450 kgCO2e/m2 However, the range between the 25th and 75th percentiles varied between building use types.
Within each building use type, the average range between lowest to highest ECI was 200 kgCO2e/m2. order & safety, higher education and assembly use types resulted in the widest ranges between lowest and highest ECI results.
Order & safety (n=3) buildings demonstrated the highest ECI values (over 700 kgCO2e/m2). We associated this with the unique design conditions and requirements of these building use types.
Several office buildings demonstrated some of the lowest ECI results, below 250 kgCO2e/ m2. We associated this with greater design flexibility within the structure and enclosure, from high-performing mass timber offices to traditional concrete. It’s important to note that office building use types also represented the most common use type within our study which may have contributed to the wider spread.
MEAN GWP INTENSITY OF NEW CONSTRUCTION PROJECTS, STRUCTURE AND ENCLOSURE
Projects by Building Use Type and Structural System Type
Assembly (Civic)
Assembly (Rec./Community)
Assembly (Stadium/Arena)
Education (Higher Education)
Education (K-12 School)
Healthcare (Inpatient)
Healthcare (Outpatient)
Laboratory (Academic)
Laboratory (Commercial)
Lodging (Hotel/Student)
Lodging (Multi-family)
Office
Other (Data Center)
Other (Order & Safety)
Figure 13: Mean GWP Intensity of New Construction Projects, Structure and Enclosure, Projects by Building Use Type and Structural System Type (kg CO2eq/m2), Life Cycle Stages A1-A3, excluding biogenic carbon
Structure GWP Intensity by Structural System Type
In Figure 14A, we investigated the ECI of our projects across six different structural system types. Most were either concrete or steel superstructures (vertical and horizontal support systems).
The mean ECI for both concrete and steel structural systems were between 250-275 kgCO2e/m2.
50% of the results (between 25th and 75th percentiles) of both structural systems are approximately between 180-330 kgCO2e/m2. It’s important to re-iterate that our methodology was focused on quantities alone and removes product-level variations for concrete and steel. Including product-specific environmental product declarations (EPDs) would widen the range of concrete structural system impacts. The wide range of steel structural systems similarly indicated a large variation in how steel structures are designed.
Mass timber versus concrete or steel: In our study, mass timber structural systems were on average over 30% lower than comparable concrete or steel structures before accounting for biogenic carbon. This is expected as mass timber does not require an energy intensive manufacturing process and is significantly lighter than steel or concrete. It is important to note that procurement for mass timber must be taken into consideration. Ecological or biodiversity impacts from the planting, harvesting, and transportation of raw timber are not captured in carbon assessments or EPDs. Ecological impacts can outweigh the embodied carbon savings if not procured responsibly.
Results in this diagram do not include adaptive reuse projects.
MEAN GWP INTENSITY OF NEW CONSTRUCTION PROJECTS, STRUCTURE
Projects by Structural System Type
Concrete
Concrete and Masonry
Hybrid (Concrete and Steel)
Hybrid (Timber with Concrete and/or Steel)
GWP Intensity (kg CO2e / m2)
Figure 14A: Mean GWP Intensity of New Construction Projects, Structure / Projects by Structural System Type (kg CO2eq/m2), Life Cycle Stages A1-A3, excluding biogenic carbon, Reuse projects not included
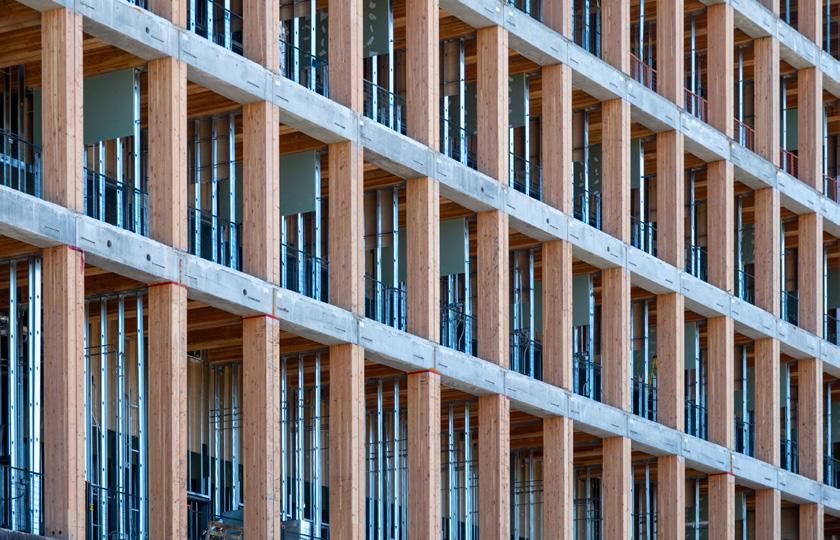
Mass Timber Steel
Right:
University of British Columbia Gateway Vancouver, BC
Credit: Michael Elkan Photography
Substructure and Superstructure GWP by Structure Type
Figures 14B and 14C expand upon Figure 14A by showing both substructure and superstructure ECIs separately.
The mean superstructure ECI of concrete and steel system types similarly shows little variation. Steel structural systems demonstrated a wider range from low to high ECI values when compared to a concrete structural systems.
The substructure results follow the same pattern as above with relatively similar mean ECIs. It is important to note that while this graph is categorized into different structural systems., the results in Figure 14C are primarily impacts from concrete as it is the predominant material for foundations and below-grade structures. Interestingly, concrete structural systems resulted in a wider range for substructure, whereas steel structural systems exhibited a narrower ECI range, opposite to the superstructure results.
Mass timber structural systems demonstrated the lowest mean ECI values of the six systems and two of the lowest superstructure ECIs despite not including biogenic carbon.
Again, mass timber procurement must be an important consideration. Ecological or biodiversity impacts from the planting, harvesting, and transporting raw timber are not captured in carbon assessments and can outweigh the embodied carbon savings if not procured responsibly.
MEAN GWP INTENSITY OF NEW CONSTRUCTION PROJECTS, SUPERSTRUCTURE
Projects by Structural System Type
Concrete
Concrete and Masonry
Hybrid (Concrete and Steel)
Hybrid (Timber with Concrete and/or Steel)
Mass Timber Steel
Intensity (kg CO2e / m2)
Figure 14B: Mean GWP Intensity of New Construction Projects, Superstructure / Projects by Structural System Type (kg CO2eq/m2), Life Cycle Stages A1-A3, excluding biogenic carbon, Reuse projects not included
MEAN GWP INTENSITY OF NEW CONSTRUCTION PROJECTS, SUBSTRUCTURE Projects by Structural System Type
Concrete
Concrete and Masonry
Hybrid (Concrete and Steel)
Hybrid (Timber with Concrete and/or Steel)
Mass Timber Steel
Figure 14C: Mean GWP Intensity of New Construction Projects, Substructure / Projects by Structural System Type (kg CO2eq/m2), Life Cycle Stages A1-A3, excluding biogenic carbon, Reuse projects not included
Substructure GWP by Foundation Type
ECIs for various substructure or foundation types also demonstrated relatively similar mean results of approximately 50 kgCO2e/m2. Figure 15 indicates that both pile foundations and shallow foundations have very similar results with the 25th to 75th percentile for deep foundations slightly lower than shallow foundations.
It’s important to note that there are several factors that influence the substructure foundation type of a system (e.g. seismic, soil type and height). In addition, there were only five buildings in the study with a deep foundation over 50 ft, while there were 23 buildings with a deep foundation under 50 ft. This could skew the results shown. Deep foundations also included several outliers.
MEAN GWP INTENSITY OF NEW CONSTRUCTION PROJECTS, SUBSTRUCTURE
Projects by Foundation Type
Deep foundation < 50 (15m)
Deep foundation > 50 (15m)
Other Foundation System
Shallow foundation
Figure 15:Mean GWP Intensity of New Construction Projects, Substructure / Projects by Foundation Type (kg CO2eq/m2), Life Cycle Stages A1-A3, excluding biogenic carbon, Reuse projects not included
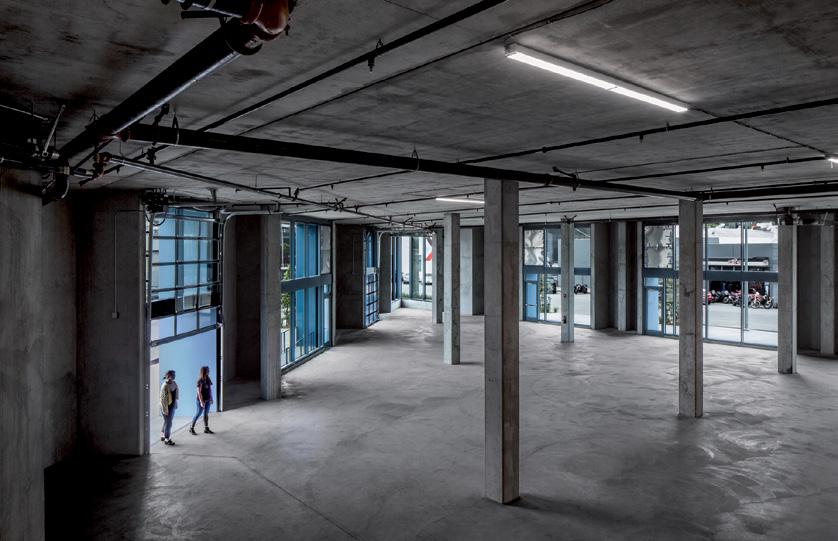
Right:
1 De Haro San Francisco, CA
Building Geography, Height, and Area
Climate Zone
Despite the fact that our projects covered nine different climate zones, the study did not find a correlation between climate zone and embodied carbon. We attributed this to the wide variety of enclosure designs and thermal performance requirements. In addition, as structure impacts dominate the ECI values, such that variations in envelope performance are less likely to impact total building ECI.
This reinforces the recommendation that with the selection of the right insulation material, improvements to passive envelope performance over the building’s lifespan should be prioritized over the additional embodied carbon from added insulation. However, we recognize that there is a point of diminishing returns and recommend that the optimal insulation levels should be best determined by a parametric energy model.
Climate Zone
MEAN GWP INTENSITY OF NEW CONSTRUCTION PROJECTS, ENCLOSURE ONLY Projects by Climate Zone
Zone 1A (Very Hot Humid)
Zone 2A (Hot Humid)
Zone 2B (Hot Dry)
Zone 3A (Warm Humid)
Zone 3B (Warm Dry)
Zone 3C (Warm Marine)
Zone 4A (Mixed Humid)
Zone 4C (Mixed Marine)
Can. 4 (<3000 HDD)
Zone 5A (Cool Humid)
Zone 5B (Cool Dry)
Zone 6A (Cold Humid)
Zone 6B (Cold Dry)
Zone 7 (Very Cold)
Zone 8 (Subarctic/Arctic)
GWP Intensity (kg CO2e / m2)
Figure 16: Mean GWP Intensity of New Construction Projects, Enclosure / Projects by Climate Zone (kg CO2eq/m²), Life Cycle Stages A1-A3, excluding biogenic carbon
Stories Above Grade
This study did not find a strong connection between stories above grade and building GWP. The dataset consisted primarily of buildings between 2-10 stories, and the findings showed that mean values fell between 300-350 kgCO2e/m2, a relatively tight range. The study found that lowrise (1-story) buildings had a wider ECI range, between 150–550 kgCO2e/m2
The results suggested that design decisions tend to have a stronger impact on ECI in low rise buildings (compared to taller buildings); however, this trend does not appear to be consistent across all structural systems. (See Structural Systems section.)
High-rise buildings with extensive elevator cores were not studied in this report and should be part of future investigations.
MEAN GWP INTENSITY OF NEW CONSTRUCTION PROJECTS, STRUCTURE AND ENCLOSURE
Projects by Stories Above Grade
Figure 17: Mean GWP Intensity of New Construction Projects, Structure and Enclosure / Projects by Stories Above Grade (kg CO2eq/m2), Life Cycle Stages A1-A3, excluding biogenic carbon, Reuse projects not included
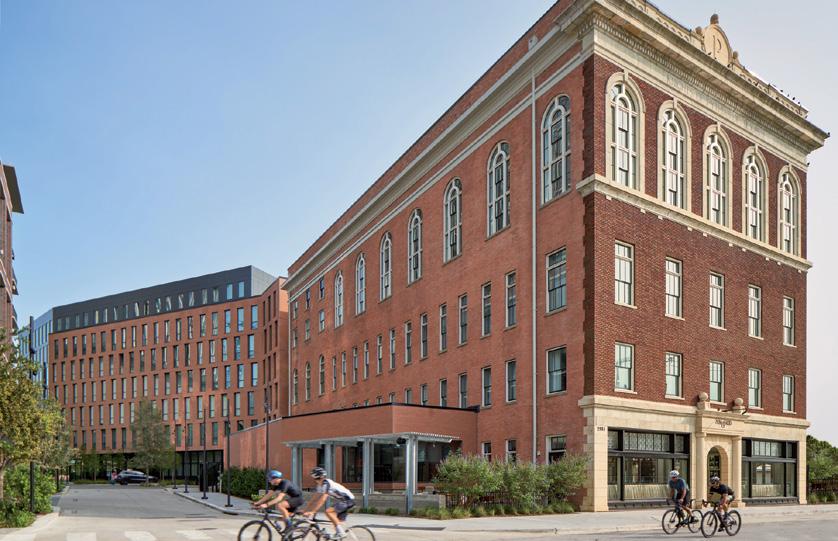
Right: Deep Ellum Hotel Dallas, TX
Building Area
This study did not find a strong connection between building area and GWP. It’s important to note that the dataset consisted primarily of projects smaller than 50,000 m2 (538,000 sf).
Mean ECI values fell between 300–400 kgCO2e/m2 but we did not find a trend that suggested area dictated building GWP. Healthcare, laboratories, and order & safety, tended to have higher GWP intensity regardless of floor area size, potentially driven by structural demands and enclosure complexity.
MEAN GWP INTENSITY OF NEW CONSTRUCTION PROJECTS, STRUCTURE AND ENCLOSURE
Projects by GFA Group
50,001+
20,001-50,000
10,001-20,000
5,001-10,000
1,001-5,000
0-1,000
Figure 18: Mean GWP Intensity of New Construction Projects, Structure and Enclosure / Projects by GFA Group (kg CO2eq/m²), Life Cycle Stages A1-A3, excluding biogenic carbon, Reuse projects not included
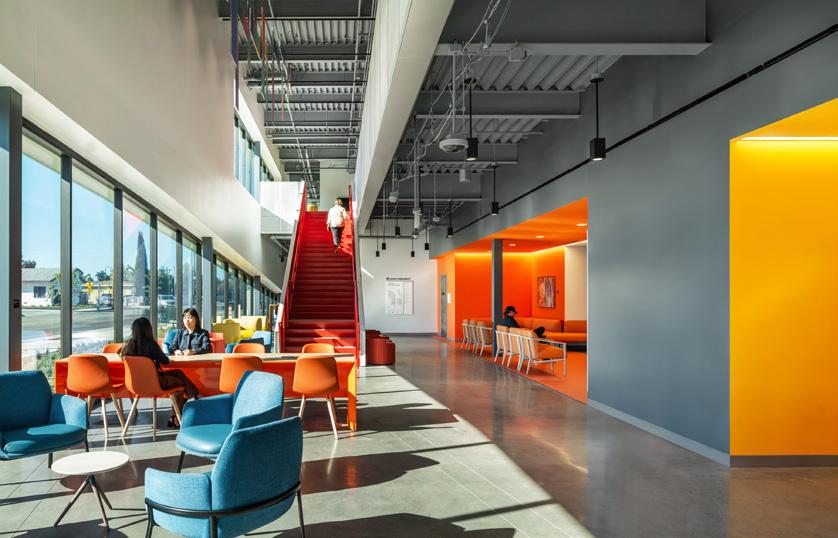
Right: Kaiser Permanente Watts Pavilion Los Angeles, CA
Adaptive Reuse
Adaptive reuse and transformation projects consistently demonstrate some of the lowest GWP intensities, reinforcing the notion that the greenest building is the one we already have. This holds true even when compared to mass timber structural systems, which still require new concrete and steel elements. This underscores that reusing any portion of the existing structural “skeleton” is the best strategy for immediate carbon reduction.
Our study also demonstrated that savings from adaptive reuse generally relate to the percentage of floor area with existing structure that is retained. Retaining 50% of a building’s floor area and associated structure generally saves 30-40% of total GWP as the structure generally makes up 60-80% of total GWP.
In our study, adaptive reuse projects on average saved about 39% of total GWP when compared to equivalent new construction. Some reuse projects went even further, achieving up to 80% less embodied carbon than building new by retaining the existing envelope as well.
Adaptive Reuse
MEAN GWP INTENSITY OF TYPICAL PROJECTS, STRUCTURE AND ENCLOSURE, SHOWING IMPACT OF ADAPTIVE REUSE
Projects by Building Use Type
Core & Shell or Simple Structure
Laboratory
Mass Timber (full or hybrid)
Other New with Interiors
Other Carbon Intensive Program
Reuse or Addition Outlier
Reuse with Timber Structure
Figure 19: Mean GWP Intensity of Typical Projects, Structure and Enclosure / Projects by Unique Characteristic (kg CO2eq/m2), Life Cycle Stages A1-A3, excluding biogenic carbon
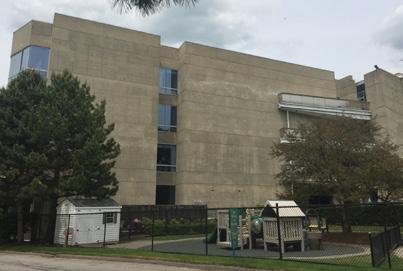
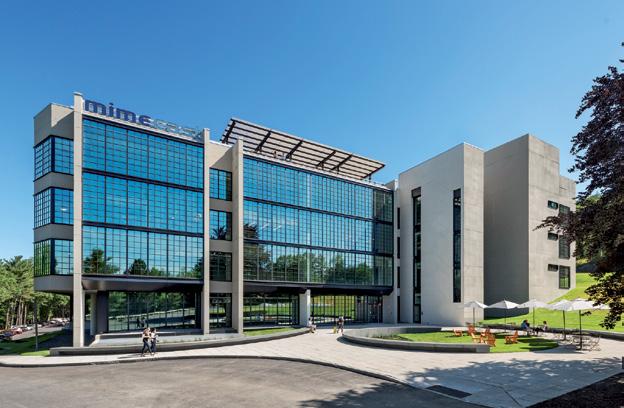
Spring Street (After)
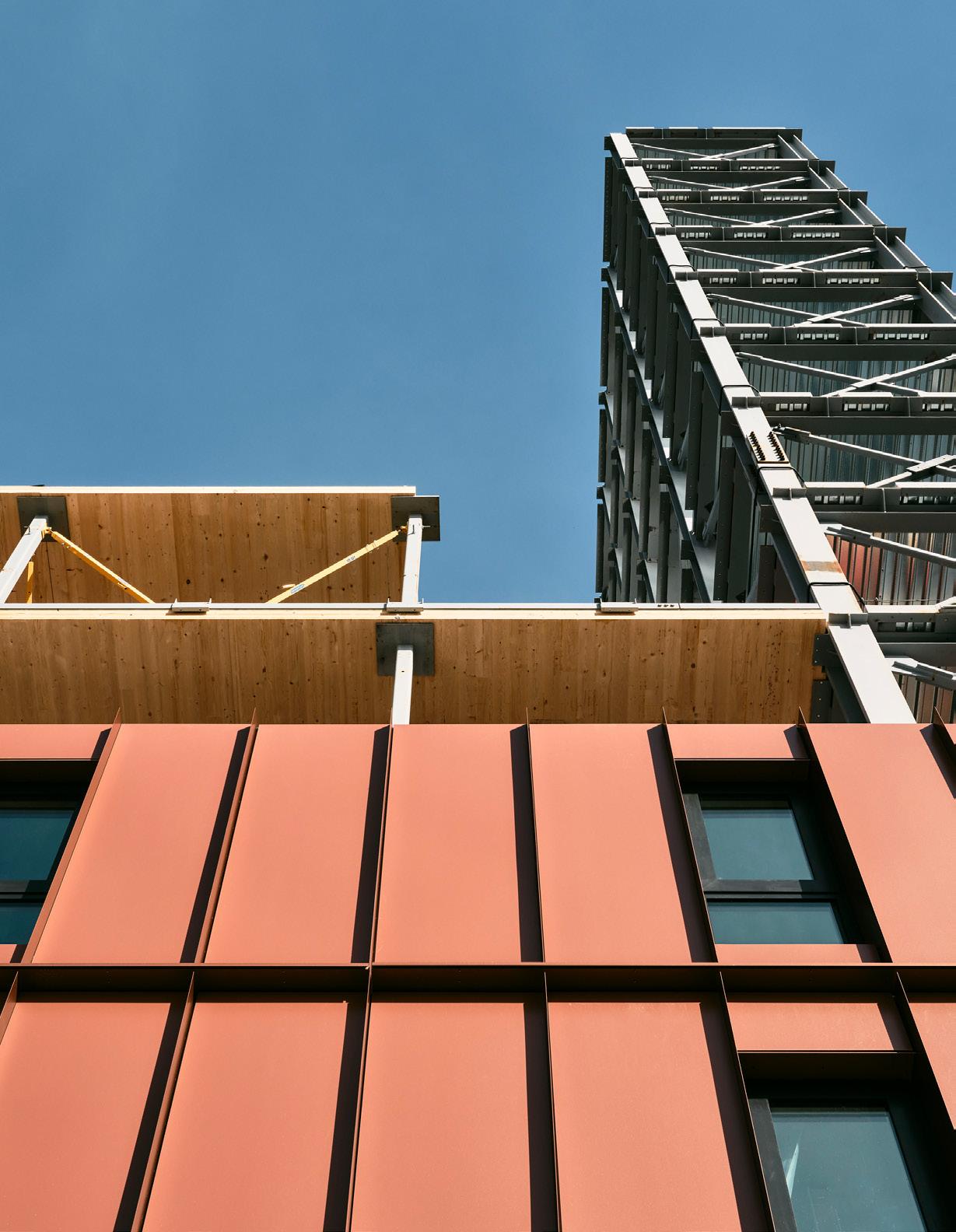
Conclusion
This extensive body of work represents a robust approach to understanding whole building embodied carbon, and the individual design elements that play a role in a project’s embodied carbon intensity. Broadly speaking, the findings confirm and align with findings from previously published embodied carbon studies. However, the large dataset of projects, along with the detailed methodology employed, sustains and supports the assumptions widely understood and reported in the architecture and design community.
It is important to note, however, that every project, regardless of building use type, structural system, or location will only see meaningful reductions in embodied carbon if a comprehensive LCA model is performed during design to test multiple iterations and design options. And LCA modeling can only serve as a useful resource in decision making if the modeling work is performed carefully by a skilled and experienced modeler. It is also important to educate the entire project team, both design and production team members, as well as consultants and the owner, on the role they play in reducing embodied carbon. Large scale decisions (such as the selection of primary structural systems or whether or not to reuse an existing building structure) are only the beginning. Thoughtful design decisions, careful specification writing, and coordination with the contractor during construction are all important elements in our quest towards decarbonization in the built environment.
Embodied Carbon Conservation Measures (ECCMs)
We strongly encourage the architecture and design community to consider the following embodied carbon conservation measures (ECCMs). These measures may help improve the GWP impacts of a projects’ building materials, construction processes, and services over its life cycle.
Structure
ECCMs
Prioritize structural material efficiency through optimized spans, grid layouts, and loadbearing wall configurations to reduce excess material use while balancing program and building use needs. Focus on high volume components first.
Implement structural efficiency techniques such as voided slabs, post-tensioning, and minimize over-engineering. For concrete, also consider adjusting cure times and mix ratios within the bounds of performance needs.
Promote hybrid systems (e.g., concrete-steel, timber-steel) for mid-rise buildings by establishing guidelines that balance material use efficiently.
Incentivize mass timber for low-to-mid-rise buildings, ensuring sustainable sourcing with FSC certification.
Prioritize shallow foundations where feasible and explore ground improvement techniques to minimize the need for deep foundations. Optimize structural loads to minimize foundation depth.
Optimize foundation designs for seismic zones using high-performance concrete with lower cement content and alternative reinforcement methods like fiber-reinforced polymers (FRPs).
Use low-carbon concrete mixes, such as those incorporating high volumes of supplementary cementitious materials (SCMs) like fly ash, slag, or glass pozzolan.
Prioritize reinforcing and structural steel made from electric arc furnaces (EAF) with high recycled content. Avoid plants located in regions that are known to be tied to a fossil fuel heavy electrical grid.
Materials
Prioritize plant-based insulation (cork, wood fiber, hemp, or cellulose), or use fiberglass or mineral wool. If rigid foam is required, specify low GWP and HFC free versions of ISO, EPS, or XPS. Avoid halogenated flame retardants.
Compare product-specific environmental product declarations (EPDs) and procure materials that comparatively demonstrate low GWP per relevant material category.
Prioritize modular construction and prefabrication for structural and envelope elements to reduce waste and GWP. Encourage high-recycled content in structural steel and concrete.
Use lightweight materials for atriums and low-carbon insulation for roofs and enclosures to offset the increased GWP from inefficient material distribution.
Geography, Climate & Scale
Consider climate-responsive materials (e.g., phase-change materials for hot climates and vacuum-insulated panels for cold climates) to reduce the need for additional insulation.
Optimize building massing to reduce wind loads. Use low-weight hybrid systems (timbersteel) to limit material intensity.
Prioritize building reuse and transformation over demolition. If new construction is unavoidable, incorporate salvaged materials from demolished buildings.
Optimize large projects’ material types, programming, and structural members through integrated collaboration with the consultant team. Consider modular, prefabricated systems that reduce waste and allow for efficient material use.
Next Steps
This benchmarking study provided a foundational framework for embodied carbon reduction strategies within Perkins&Will’s projects and contributed valuable data to industry-wide efforts toward decarbonization, including the Carbon Leadership Forum’s (CLF’s) V2 Embodied Carbon Benchmarking study and dataset, and the International Institute for Sustainable Laboratories embodied carbon benchmarking database and dashboard. Perkins&Will Research is performing additional analysis on both our dataset and CLF’s Harmonized Dataset of High-Resolution WBLCA Results and will release a follow up report with additional findings. Future efforts will expand to explore the following in greater depth:
⇒ The architecture community needs future studies that expand their scope to include:
‒ Collaboration with contractors to model realized A4-A5 (product transportation and construction/installation) impacts.
‒ Greater focus on life-cycle stages B, C, and D, with detail on service life and end of life assumptions and how these assumptions affect results.
‒ Expanded physical scope, including a more thorough study of interior partitions, finishes, and furnishings, and mechanical electrical and plumbing (MEP) systems.
‒ The impacts of refrigerants and scenarios for refrigerant leakage over time.
‒ Updated WBLCA reports to correspond with forthcoming data updates, including CLF’s 2025 Material Baselines and the Open Data for Climate initiative (ODCi) dataset (SOURCE) that will be incorporated into Tally 2.0.
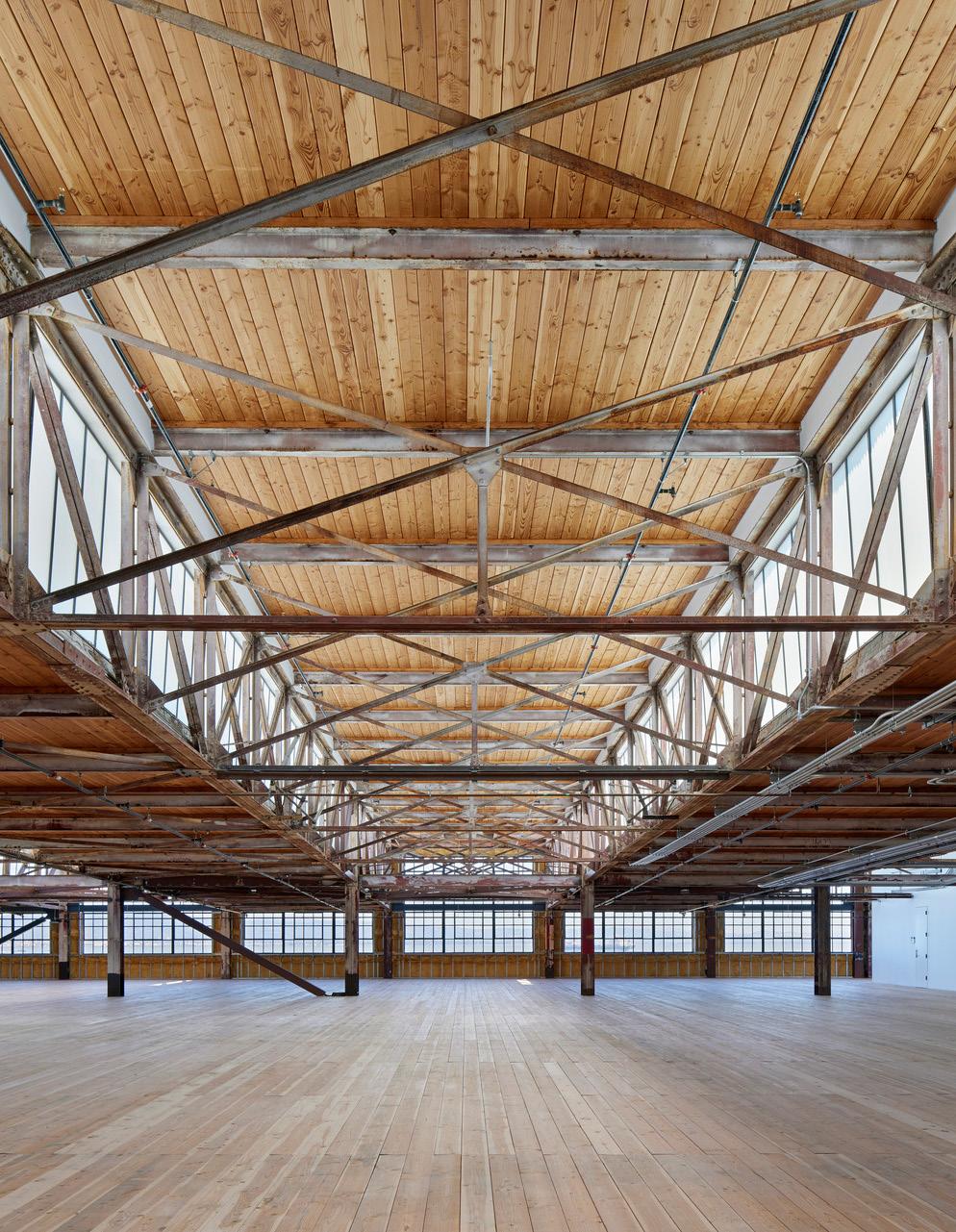
Appendix
Resources and Glossary
Resources
Carbon Leadership Forum
Carbon Leadership Forum Community
Building Transparency (the EC3 tool & tallyLCA)
Architecture 2030 Carbon Smart Materials Palette
Structural Engineering 2050 (SE 2050)
Ellen MacArthur Foundation (circular design)
Glossary
A Baseline is a base value used as a reference point for comparisons.
A Benchmark is also a point of reference but contributes to a set of reference points that examined together represent averages.
Biogenic carbon is the carbon dioxide sequestered by, stored within, and emitted from plants and biological sources (rather than fossil sources).
Carbon Dioxide Equivalent Emissions (CO2e) is the standard measure for GWP impacts (aka, “carbon”). The “e” in CO2e stands for “equivalent.” This means that many greenhouse gases are combined in a single metric by converting each GHG into an equivalent amount of carbon dioxide based on its GWP.
Carbon Intensity is a normalizing metric that represents GWP impacts divided by a common metric. For buildings, GWP is typically divided by the area of study (CO2e/ft² or m²).
Circular Design reduces waste and extraction of raw materials through design for adaptability, disassembly, and reuse.
Embodied carbon conservation measures (ECCMs) are actions to improve the GWP impacts of a projects’ building materials, construction processes, and services over its life cycle.
Embodied carbon refers to the greenhouse gas emissions (GHGs) associated with materials and services throughout their life cycles. These emissions occur through raw material supply, manufacturing, transportation, installation, use, maintenance, decommissioning, and disposal of building materials. Each material used in a product, building, site, or infrastructure project has an embodied carbon footprint.
Environmental Product Declarations (EPDs) are 3rd-party verified standardized documents that use LCA to report products’ GWP Impacts. EPDs can be “Industry Average,” combining the impacts of many products within a category, or “Product-Specific,” focusing on impacts from a single product from a single manufacturer. We use Product-Specific EPDs to pick lower GWP products.
Global Warming Potential (GWP) is a metric used to compare the global warming impacts of different greenhouse gases. GWP impacts are measured over a period of time (most commonly 100 years).
Greenhouse gases (GHGs) contribute to climate change through the “greenhouse effect” as they absorb infrared radiation.
Life Cycle Assessment (LCA) is a systematic method for calculating the environmental impacts of materials, products, services, and buildings throughout their life cycles. “Whole Building LCA” (WBLCA) calculates these impacts for a building over a reference study period.
Life Cycle Assessment Stages (LCA Stages) are the periods of a product, process, or service’s reference service life.
Operational carbon refers to the GWP impacts associated with energy used to operate equipment and buildings.
Product-Stage, or Cradle to Gate, Embodied Carbon includes only CO2e emissions that occur through LCA Stages “A1: Material Supply,” A2: Transport [of products during manufacturing],” and “A3: Manufacturing”
A Reference Study Period (RSP) is the timeframe used to assess a building in LCA. The RSP affects a building’s “Use Stage” impacts, which aggregate as the building is used. In some cases, the RSP may be equivalent to a building’s required service life. In others, the RSP may be set to a standardized value, such as 50 or 60 years, for the purposes of benchmarking or certification requirements.
Sensitivity Analysis identifies variables in a study that have the greatest impact on its results.
Uncertainty stems from both the quality of data used and the application of data. Uncertainty is quantified by probability distribution. It can also be expressed as an uncertainty factor.
Uncertainty Analysis identifies which portions of an LCA are most uncertain, combined with assessing these variable’s sensitivity to estimate confidence in study results.
Variability refers to the multiple values a quantity may have and the divergence of data from its mean value (e.g., materials’ GWP impacts vary from product to product).
Whole life carbon refers to combined embodied and operational GWP impacts of a building, measured over a reference study period.
Methodology
This study includes underlying data that has been post-processed to account for variations from productspecific reductions.
Our study follows whole building life cycle assessment (WBLCA) best practices, aligning with methodologies established by the Carbon Leadership Forum (CLF) and other industry approaches. To ensure high-resolution data accuracy, we evaluated both built projects and those in the construction administration (CA) phase, using a consistent modeling approach with TallyLCA across all assessments. The primary focus was on A1–A3 life cycle stages (raw material supply, transport, and manufacturing), as these phases account for the majority of embodied carbon emissions in new construction. However, A4–A5, B, C, and D life cycle stages were also recorded for future research. To maintain alignment with LEED, ILFI, and ASHRAE/ICC 240P,
a 60-year reference study period was applied, with default service lives for individual materials based on TallyLCA assumptions.
The physical scope of this study included sub-structure, super-structure, and enclosure, with interior components assessed only if they were modeled. Since interior scope varied across projects, some included only core & shell (C+S), while others had full interior fit-outs. In cases where interiors were included, the study accounted for finishes (ceilings, walls, flooring) and partitions (framing, insulation, and fenestration), but casework, furnishings, and MEP systems were excluded. Additionally, balconies and exterior shading devices were considered as additive components that contribute to embodied carbon but were not included in all analyses due to inconsistencies in modeling across projects.
Retaining Walls & Footings Slabs, Framing, & Reinforcement
* OTHER
nj Basements & Parking
+ ADDITIVE
nj Balconies and large decks
nj Exterior shading devices
Cladding, Insulation, Roofing, Fenestration (Doors, Glazing, & Windows)
Ceilings, Walls, & Floors Framing, Insulation, & Fenestration (Doors, Glazing, & Windows)
nj Other unique/abnormal accessories that would make the building’s BOM atypical
✗ EXCLUDED
nj Sitework
nj Mechanical, Electrical and Plumbing (MEP) Systems
nj Casework
nj Interior Furnishings, Fixtures, and Equipment (FF&E)
To ensure comparability across projects, a harmonization approach was applied to key material impact factors, particularly for concrete, steel, rebar, aluminum, and XPS insulation. Instead of using regionally specific material data, the study standardized these values by applying the NRMCA 2023 National Average for concrete mixes and assuming national average GWP values from CLF’s baselines. These adjustments help isolate architectural and structural design choices from regional material variability, enabling clearer cross-project comparisons. While biogenic carbon impacts were tracked, they were not included in the reported results, with further details provided in the Appendix. Modeling was conducted by trained designers familiar with Revit and TallyLCA, ensuring consistency in data input. However, QA/QC reviews were performed on select projects, and variations in modeling approaches, skill levels, and available material assignments introduced some data quality limitations, which are acknowledged in this study.
Methodology Scope
System Boundaries:
Environmental Impacts: The findings of this report focus solely on global warming potential (GWP), as this impact is the primary contributor to climate change. Future studies may explore additional environmental impacts quantified through LCAs. Tally LCA results calculate a set of environmental impacts, including:
nj Global Warming Potential
nj Acidification Potential
nj Eutrophication Potential
nj Smog Formation Potential
nj Non-Renewable Energy
Life Cycle Stages: This study focused on the upfront carbon impacts associated with product manufacturing; those that occur before a project’s construction is started, as these are the primary sources of lifetime emissions for new construction projects that have a concrete or steel structure. These impacts are also most directly controlled through design decisions. These Life Cycle Stages include:
nj A1: Raw Material Supply
nj A2: Transport (to factory)
nj A3: Manufacturing
The impacts from all other phases beyond A1-A3 have been recorded as these may be used in future research.
Biogenic carbon was not considered in this study.
Limitations of Existing Studies and Data
Efforts to reduce embodied carbon in the built environment have been accelerated by groundbreaking industry case studies and innovations, yet the evolving nature of LCA standards and methodologies continue to present challenges to the creation of reliable benchmarks. While LCA findings from an experienced modeler are directionally accurate, variability is introduced by differences among LCA tools, datasets, and approaches to defining materials. This variability presents challenges when looking for valid comparisons across projects. Furthermore, whole building LCAs are only directly comparable if the buildings being studied share the same location, size, and program type, so drawing conclusions across projects whose variables differ requires both a large number of studies and consistency among those studies. Several variables contribute to these inconsistencies, including:
nj Software tools and versions: Frequent updates to background life-cycle inventories impact global warming potential (GWP) values, creating discrepancies.
nj Building information modeling (BIM) accuracy: Variations in accuracy and methods for defining components within tools like Revit affect data reliability.
nj Key project metrics: Differences in approach to measuring floor area, façade area, grid spacing, and other parameters create variability.
nj Multiple reference standards: The use of different benchmarks, such as Carbon Leadership Forum (CLF) V1 or V2, Structural Engineers 2050 Challenge (SE2050), and the Royal Institution of Chartered Surveyors (RICS) Framework, complicates cross-comparison.
While tools like the Embodied Carbon in Construction Calculator (EC3) and the proliferation of Environmental Product Declarations (EPDs) have made it easier to understand the environmental footprint of specific products, they do not address how architectural and structural design decisions influence a building’s overall carbon footprint. This challenge is analogous to selecting the most efficient HVAC system without first analyzing the building’s energy demand.
Model Accuracy
To address industry challenges with inconsistent reporting and LCA methodologies, our internal study focused on controlling variables and refined data to enhance consistency and accuracy.
Key elements of our methodology include:
• Model Accuracy: We studied only built projects or those in the Construction Administration phase (2023–2024). Each project used BIM models designed to a construction or contract level, ensuring high model definition.
• Harmonized Definitions: The outputs of WBLCAs are entirely dependent on the material definitions assigned to each building component. In many cases, the material definitions are dependent on the region the material was procured in. In the case of structural materials that make up the majority of a building’s volume or weight, material definitions can shift a building’s embodied carbon footprint dramatically. To address this, we applied harmonized material definitions for concrete, reinforcing steel, concrete, reinforcing steel and XPS insulation across the entire dataset. This limits product-specific variations due to location and is a unique factor to our study. By harmonizing our major material definitions, we can better identify the building design choices that result in higher or lower embodied carbon.
• Core Physical Scope: We concentrated on architectural, structural, and interior scopes to minimize external variability. This narrower focus allows clearer insights into how design decisions affect embodied carbon.
Due to these focus areas, the results from our study are not comparable with other large databases or reports.
Limitations & Disclaimers
Scope:
nj The dataset was limited to Perkins&Will projects that met specific completeness criteria. While substantial, it’s not fully representative of our entire portfolio.
nj Some building types (e.g., outpatient healthcare, arenas, stadiums, hotels, student housing, and order & safety) were underrepresented, and certain typologies are not included at all.
nj Projects were heavily concentrated in the U.S. Northwest, so not all climate zones or building code variations are captured.
nj Not all projects included interior work; varying modeling approaches further affect interiors data consistency.
nj Projects ranged from reuse to core and shell to new construction with full interiors, with diverse structural systems. This limits the precision of use type-specific analyses when sample sizes are small.
nj Sitework, mechanical, electrical, and plumbing (MEP), casework and furnishings were excluded from this study.
Data Quality:
nj Volumes and component details vary due to different Revit modeling approaches, level of detail, and potential over/ under sizing of elements.
nj Inputs rely on individual modelers’ data entry and quality control checks.
nj Inputs relied on available “assignment” options via TallyLCA. Not all options covered in the drawings and specifications were available.
nj Team members had varying proficiency with Revit and TallyLCA. Training was provided, but skill levels still differed.
nj Some teams were able to engage with their structural engineers to verify definitions of structural elements, not all.
nj QAQC was performed by the research team on some projects, not all, to address obvious abnormalities.
Future Studies Identified
As the abilities to measure the embodied carbon of our projects continues to evolve, several areas of further study have been identified to refine benchmarks, improve modeling methodologies, and expand the scope of impact reduction strategies. These future research directions will provide more robust data to inform sustainable design decisions and drive meaningful reductions in embodied carbon across our portfolio.
1. Interiors: This study primarily focused on structural and envelope components, but interior materials contribute significantly to embodied carbon due to their shorter life spans and frequent replacement cycles. Future research will:
‒ Analyze the embodied carbon impact of common interior materials, finishes, and furnishings.
‒ Establish benchmarks for interior design strategies that reduce embodied carbon without compromising functionality or aesthetics.
‒ Explore circular economy principles, including design for deconstruction, material reuse, and refurbishment strategies.
2. Biogenic: The role of biogenic carbon storage in mass timber and other bio-based materials was not included in this study. Future analysis will:
‒ Evaluate different biogenic carbon accounting methodologies and their implications for wholebuilding LCA results.
‒ Investigate the long-term carbon storage potential of timber, bamboo, hempcrete, and other emerging bio-based materials.
‒ Provide guidance for responsible procurement and certification of biogenic materials to ensure sustainability and carbon sequestration integrity.
3. Additional Life Cycle Stages: This study focused on A1–A3 (upfront carbon) as they account for the majority of a building’s lifetime emissions. Expanding the scope to include additional life cycle stages will allow for a more comprehensive assessment:
‒ A4–A5 (Transport & Construction Process): Investigating transportation emissions for different supply chain scenarios and emissions from construction-site activities.
‒ B (Use Phase): Evaluating emissions from maintenance, repair, replacement, and potential operational synergies between embodied and operational carbon.
‒ C–D (End-of-Life & Reuse Potential): Assessing deconstruction strategies, material recovery rates, and the carbon savings potential from reuse and recycling.
4. Embodied Carbon Conservation Measures (ECCMs)
Development: This study highlighted the impact of design decisions on embodied carbon. Further research will:
‒ Develop specific ECCMs tailored to different typologies and structural systems.
‒ Establish clear implementation guidelines for designers, engineers, and procurement teams.
‒ Quantify the impact of key ECCMs, such as lowcarbon concrete mix designs, high-recycled content materials, and optimized structural grids.
5. Building Use Type Deep Dive: While this study established baseline embodied carbon trends across typologies, deeper investigations are needed for highimpact building types. Future research will:
‒ Conduct detailed analyses of healthcare, laboratory, and civic buildings, which exhibited higher GWP intensities.
‒ Explore best practices for optimizing design decisions specific to each use type.
‒ Evaluate alternative structural and enclosure systems that could lead to significant carbon reductions.
6. Additional typologies: The dataset underrepresented certain building types, limiting broader industry applicability. Future benchmarking studies will:
‒ Expand the database to include typologies such as stadiums, data centers, industrial facilities, residential projects, and transportation infrastructure.
‒ Investigate the embodied carbon impacts of prefabricated and modular construction techniques.
‒ Examine trends in mixed-use developments and their embodied carbon trade-offs across multiple programs.
7. Seismic and Climate Adaptation Strategies: Climate resilience and seismic design considerations significantly influence embodied carbon. Future research will:
‒ Analyze the relationship between embodied carbon and high-seismic design requirements across different structural systems.
‒ Investigate the carbon implications of designing for extreme weather events, including high-wind and flood-prone regions.
‒ Develop design strategies to balance resilience, durability, and embodied carbon reduction.
8. Low-Carbon Procurement and Material Innovation: Material selection remains a critical driver of embodied carbon. Future studies will:
‒ Evaluate the impact of procurement decisions on embodied carbon, including regional variations in material production and supply chain emissions.
‒ Investigate the role of Environmental Product Declarations (EPDs) and transparency in material specification.
‒ Explore emerging low-carbon materials, such as carbon-negative concrete, algae-based insulation, and geopolymer alternatives to traditional cement.
9. Integration of MEP Systems and Refrigerants: The mechanical, electrical, and plumbing (MEP) systems were not included in this study but are increasingly recognized as significant contributors to embodied carbon. Future research will:
‒ Quantify the embodied carbon of common HVAC, electrical, and plumbing systems.
‒ Assess the impact of refrigerants and leakage rates on whole-building LCA results.
‒ Provide design guidance for integrating low-carbon MEP strategies into holistic carbon reduction plans.
10. Circular Economy and End-of-Life Scenarios: The potential for material reuse and recycling at the end of a building’s life can greatly influence total embodied carbon. Future studies will:
‒ Examine the feasibility of design for disassembly strategies to maximize material recovery.
‒ Investigate real-world case studies on building material salvage and reuse.
‒ Develop frameworks for assessing carbon savings from adaptive reuse and deconstruction approaches.