
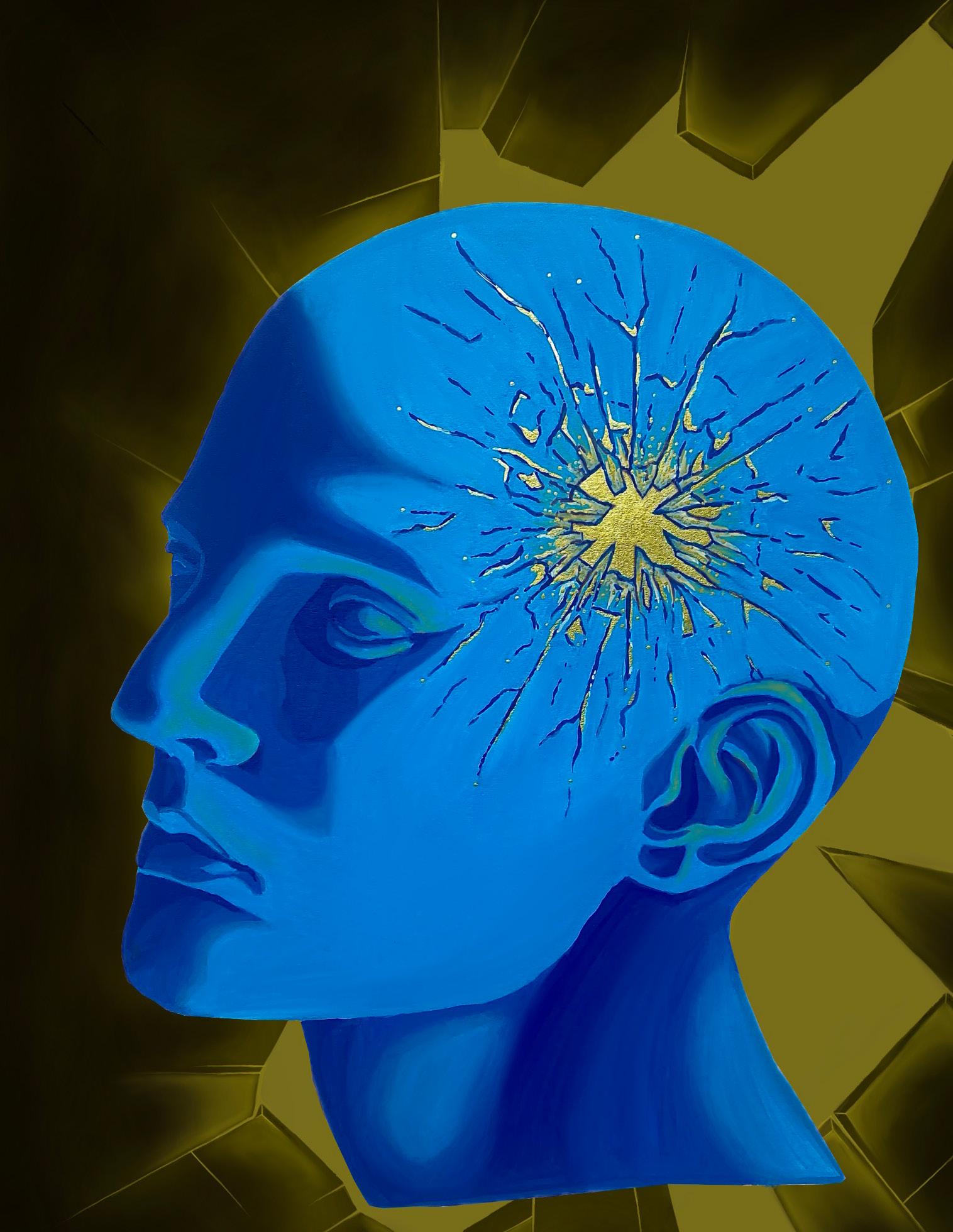
Healing Through Hormones: The Neurobiological Effects of Hormone Replacement Therapy
Harnessing the Heat: Nature’s Fiery Solution to Pain
The Future of Thought: Neuralink and Brain Computer Interfaces
10TH ANNIVERSARY OF GREY MATTERS JOURNAL AT VASSAR COLLEGE: FACULTY PROFILES
FEATURED ARTICLE
art by Dylan Berman & Anna Bishop 14 19 24 29 8
HEALING THROUGH HORMONES: THE NEUROBIOLOGICAL EFFECTS OF HORMONE REPLACEMENT THERAPY
by Michael Silva | art by Elsie McKendry
UNDERCOOKED AND OVERLOOKED: WHEN WORMS INFEST THE BRAIN
by Jack Matter | art by Lola Yost
FROM HEALING DEITIES TO HEALTHCARE DIFFICULTIES: THE STRUGGLE TO UNDERSTAND FUNCTIONAL SOMATIC DISORDERS
by Lucia Holbrook-Brown | art by Ansen Chamberlain
FEATURED ARTICLE
HARNESSING THE HEAT: NATURE’S FIERY SOLUTION TO PAIN
by Kaitlin Raskin | art by Mischa Landgarten & Iris Li
TOTAL BLITZ ON THE BRAIN: THE ELUSIVE TACTICS OF CHRONIC TRAUMATIC ENCEPHALOPATHY
by Daniel Bader | art by Alexandra Adsit
10TH ANNIVERSARY OF GREY MATTERS JOURNAL AT VASSAR COLLEGE: FACULTY PROFILES
‘ONLY LOVE CAN HURT LIKE THIS’: UNDERSTANDING BROKEN HEART SYNDROME by Chloe Bilger | art by Elizabeth Catizone
FROM THE SHADOWS: THE HIDDEN CONSEQUENCES OF BLACK MOLD
by Layla Fakki | art by Emily Holtz
CAN MUSIC TEACH US HOW TO TALK? MUSIC’S ROLE IN SPEECH THERAPY
by Leo Mahlke | art by Racine Rieke
A MELANCHOLIC TUNE: THE DYNAMICS OF NEUROINFLAMMATION AND DEPRESSION by Thomas Doyle | art by Ava Sclafani
SHADES OF CARE: RACIAL DISPARITIES IN PAIN TREATMENT
by Anoushka Bhatt | art by Nancy Duer
FEATURED ARTICLE
THE FUTURE OF THOUGHT: NEURALINK AND BRAIN COMPUTER INTERFACES by Daniel Wunschel | art by JD Jaromilek
Art by Alexandra Adsit
If you have any questions or comments regarding this Issue 10, please write a letter to the editor at brainstorm.vassar@gmail.com.
Check out our website to read our articles, find out how to get involved, and more at greymattersjournalvc.org.
Senior Managing Editor & Treasurer
Senior Editor, Scientific Review
& Website Manager
Scientific Review
Outreach Coordinator
Assistant Layout Executive
Alexandra Adsit
Ansen Chamberlain
Ava Sclafani
Dylan Berman
Elizabeth Catizone
Elsie McKendry
Emily Holtz
JD Jarolimek
Lola Yost
Mischa Landgarten
Nancy Duer
Racine Rieke
Abigail Tramell
Ashley Hong
Cailey Metter
Caroline Martin
Dimple Kangriwala
Eden Lanham
Giana Rizzo
Hannah Lee
Katerina Hristova
Krisha Jeevarathnam
Matthew Rawson
Maxx Martinez
Naomi Meyers
Nidhi Pandruvada
Nika Jalali
Paige King
Sarah Boucher-Rowe
Shaylee Bonsness
Tabitha Schully
Talia Mohideen
Talia Roman
Anoushka Bhatt
Chloe Bilger
Daniel Bader
Daniel Wunschel
Jack Matter
Kaitlin Raskin
Layla Fakki
Leo Mahlke
Lucia Holbrook-Brown
Michael Silva
Thomas Doyle
Alexandra Astalos
Alma Sutherland-Roth
Ashton Spradling
Bailey Mann
Bertha Shipper
Eli Kanetsky
Emily Nothdurft
Evan Seker
Jadyn Smith
Joseph Lippman
Kate Billow
Lucy Gaffneyboro
Malathi Kalluri
Ren Nicolau
Hadley Bergstrom PhD
Lori Newman PhD
Sue Trumbetta PhD
Lauren Gracie - Layout
Amelie Grube
Anisha Azizi
Arden Spehar
Claire Bennett
Erin Thatcher
Grace Cabasco
Jacqueline Rosenblum
Jenais Panday
Julia Fallon
Kay Tichy
Kyle Benson
Lauren Gracie
Lea Repovic
Lena Lynch
Lila Horberg Decter
Lily Paine
Maryam Basma Sultan
Neha Dhakal
Nico Silverman-Lloyd
Owen Raiche
Quincey Dern
Sadie Bakken-Durchslag
Stephanie Norris
Susanna Osborne
Tyler Lawton
Zachary Cahn
Zachary Garfinkle
Zayn Cheema
Zoe Rodriguez
I didn’t come to science communication thinking it would be an act of resistance. I came to it because I loved science and wanted to talk about it with the people around me. But it didn’t take long to realize that, in a world where truth is so often distorted, and where misinformation spreads faster than facts, making science understandable and accessible is not just important. It’s urgent.
We’re in the middle of a troubling shift, where scientific consensus is questioned not on the basis of evidence, but on the basis of political agendas. The distortion of truth doesn’t live in the shadows — it’s front and center, broadcast widely, dressed up as skepticism. In this environment, doing good science isn’t enough. Publishing papers is not enough. If our discoveries never leave the walls of academia, if our language stays coded and exclusive, then we’ve left the public — and the truth — vulnerable. At Grey Matters, our work has always been rooted in the idea that science is for everyone. Because we believe that understanding science shouldn’t require a PhD or fluency in jargon. It should just require curiosity. Grey Matters Journal exists to serve as a bridge between science and society. Our responsibility is not just to inform, but to empower, so that facts remain stronger than fear, and discovery remains a force for good.
Over my time with Grey Matters, I’ve come to appreciate just how much labor goes into building the bridge. It means writing with clarity, but also with empathy, humor, and humility. It means constantly asking ourselves: who’s being left out of this conversation, and how can we bring them in? There have been moments when this work felt overwhelming. But what’s kept us going, what keeps me going, is this stubborn optimism that if we make science more transparent, more human, and more joyful, people will show up for it.
To our readers: thank you for continuing to show up with curiosity, issue after issue. In this tenth edition of Grey Matters Journal at Vassar College, we’re excited to share eleven thoughtfully crafted articles exploring the complexities of the brain from a wide range of perspectives. We’re also thrilled to mark this milestone issue with something special. For the first time, we’ve invited some of our esteemed faculty members to share their own reflections on what drew them to neuroscience, what accessibility in science means to them, and what questions keep them curious in their labs. Their voices add depth to this issue and remind us that science is, at its core, a human pursuit.
As I step down from my role, I’m filled with gratitude. For the team that made this journal a living, breathing thing. For the late-night edits, the brainstorming sessions that turned into breakthroughs, and the laughter we somehow always found in the margins. Most of all, I’m grateful for the collective courage it takes to challenge the way things have always been done. Although this goodbye is bittersweet, I am confident in the leadership and vision of Evelynn Bagade, who will be stepping into the role of Editor-in-Chief. Evelynn’s creativity and commitment to making science more accessible have already strengthened this journal in countless ways, and I know she’ll continue to lead with the same intention and heart that make this work matter. Though my time as Editor-in-Chief is ending, our mission will stay with me. It’s a cause I will carry forward, as we all must, in the face of mounting challenges to the integrity of scientific discourse.
At the end of the day, we’re not just publishing science. We’re creating space for dialogue, for dissent, for discovery. And that feels like something worth fighting for.
With deep appreciation and hope,
Shawn Babitsky Editor-in-Chief
by Michael Silva | art by Elsie McKendry
Have you ever felt uncomfortable in your own skin? For most people, this feeling might be fleeting — a momentary discomfort, easily shaken off — but for some individuals, the sense of disconnect between body and gender identity can be both profound and persistent. By and large, sex and gender refer to two concepts that, while often connected, are differentiable [1, 2, 3]. Gender generally refers to socially constructed roles, behaviors, and expectations, whereas sex encompasses biological characteristics such as chromosomes and reproductive anatomy [2, 3, 4]. When an individual’s gender identity divergesw significantly from their assigned sex at birth, they may experience gender dysphoria (GD) — a clinically recognized condition marked by distress, discomfort, and a profound sense of incongruence between physical appearance and internal identity [3]. GD is explicitly recognized in the standard American diagnostic manual, whereas other international diagnostic manuals emphasize the broader concept of gender incongruence (GI), focusing less on distress and being more inclusive of the diversity of transgender experiences [5]. Although GD is commonly associated with GI, debate continues within the medical community about whether distress must necessarily accompany incongruence [6].
When treating GD and GI, the discussion often centers around hormones, the chemical messengers that travel through our bloodstream to produce diverse effects throughout the body [7]. Sex hormones produced by the gonads — ovaries in females and testes in males — influence physical development as well as mood, cognition, and emotional regulation [8, 9]. Sex hormones play a crucial role during puberty and continue to exert effects throughout one’s lifespan [9, 10]. Among transgender individuals, hormone replacement therapy (HRT) is a widely prescribed and medically recognized intervention for alleviating GD, allowing one’s body to align more closely with their gender identity [11, 12, 13]. HRT improves overall psychological well-being, with a significant reduction in depression, anxiety, and suicidality in transgender populations [13, 14, 15]. Despite clear clinical benefits, gender-affirming care remains a politically fraught issue [16]. Recent efforts to restrict HRT and puberty blockers, such as federal bans on research funding or laws denying access, threaten the availability of care and undermine public understanding of its medical legitimacy, especially for transgender youth [16, 17, 18]. In the face of mounting misinformation, examining the biological and psychological evidence supporting HRT is not only necessary but urgent. Understanding how hormones and HRT affect the body and brain affirms the importance of transgender healthcare, reinforcing its legitimacy as a medical intervention.
Any discussion of HRT begins with a closer look at estrogen and testosterone, hormones present in all humans and virtually all vertebrates, regardless of sex [19, 20, 21]. Estrogen is essential for female sexual development during puberty, such as breast growth and the regulation of the menstrual cycle [22, 23]. Testosterone is typically associated with its role in the development of male sexual characteristics, such as voice deepening and facial hair growth [24]. Additionally, testosterone belongs to a class of hormones called androgens, substances capable of developing and maintaining masculine characteristics in reproductive tissues [25]. Estrogen and testosterone together play a critical role in the overall health of both sexes beyond puberty and sex differences [26, 27]. For example, estradiol, the predominant form of estrogen during reproductive years, is central to maintaining bone density and cardiovascular health in women [27, 28]. Testosterone also enhances bone mineral
density in women by increasing the presence of estradiol [27]. In men, a portion of testosterone is converted to estradiol, which is crucial for male bone strength and cardiovascular functioning [27]. Independently, testosterone has an active role in supporting physiological processes like fat distribution [29]. In both sexes, free-floating testosterone and the activation of androgen receptors are positively correlated with muscle mass and strength [30]. The metabolism, bone health, and overall physiological functioning of males and females heavily rely on specific proportions of both estrogen and testosterone [26].
Beyond their effects on the body, sex hormones also influence brain chemistry by interacting with neurotransmitters — chemical messengers that carry signals across the gaps between neurons called synapses [31]. Testosterone and estrogen act as neuromodulators, a class of signaling molecules that regulate neurotransmitter systems [31]. Estradiol boosts neurotransmitter production, including serotonin, associated with mood, and dopamine, associated with reward [31, 32, 33]. Estrogen can help reduce symptoms of anxiety and depression by increasing serotonin synthesis and receptor activity [31, 34, 35]. Estrogen improves dopamine signaling in brain regions that control emotion and reward through similar mechanisms [34]. Furthermore, estradiol promotes synaptic plasticity, or the ability of neurons to change the strength and quantity of their synaptic connections over time [36]. Estradiol also modulates glutamate, the major excitatory neurotransmitter, which in turn mediates the synaptic plasticity essential for learning and memory [31, 37]. The promotion of synaptic plasticity and increase in serotonin and dopamine signaling may help explain why many transgender women report improved mood and mental well-being after beginning estradiol as a form of HRT [13, 38, 39]. Like estrogen, testosterone influences mood and brain function in both sexes, but its effects can differ significantly [31, 40, 41]. Whereas estrogen generally increases dopamine activity in reward pathways, high testosterone levels can suppress these pathways [37, 42]. Although testosterone and estrogen have some contrasting effects on the brain, they are not necessarily opposites; the hormones can have complementary, balancing roles in regulating the brain’s chemistry [37, 42]. Therefore, understanding the effects of sex hormones is crucial, as they may constitute a biological basis for the mood and cognitive changes associated with HRT [43].
The notion that male and female brains are fundamentally different due to the developmental influences of sex hormones has long been ingrained in public perception and continues to elicit scientific debate [1, 44]. While there are some measurable sex differences in brain structure, many are subtle and do not contribute to functional disparities [1, 45]. Human brains exist on a continuum shaped by hormonal exposure, genetics, and environmental factors [44, 46]. While testosterone and estrogen do play a role in shaping brain development, their effects are often more complex than a simple binary division, especially considering the varying levels of each hormone in both sexes [47, 48]. In reality, male and female brains are mostly similar, with only a small portion of the population that displays substantial sex differentiation between their brains [47]. Emerging research on brain plasticity and the effects of HRT further challenges the assumption of rigid male-female brain distinctions, highlighting that while hormones do influence brain structure and function, they do not operate within a sexual binary [49, 50, 51].
One of the most widely discussed differences between male and female brains is size [52]. Certain regions of male brains tend to exhibit denser gray matter, areas primarily containing neuronal cell bodies [53]. Cisgender women show a lower total gray matter volume and a thinner cortex, the outer layer of the brain [41, 53]. On average, male brains are about 11% larger than female brains, but differences are proportional to body size and do not correlate with cognitive ability [41, 44]. Additionally, the amygdala, a major processing center for emotions, was previously thought to be larger in males due to its role in aggression [54]. However, there are no statistically significant sex differences in the amygdala after controlling for overall brain size [54]. The same is true for the hippocampus, a structure involved in memory that was historically reported as larger in females [55]. Ultimately, absolute differences in brain size are not inherently meaningful, especially regarding the brain’s functional capacity [56, 57].
The belief that there is a sex binary in the brain is further disputed by studies on transgender brains. Even before HRT, some transgender individuals seem to show sex-atypical features in brain structure and connectivity that may be indicative of differences in
neurodevelopment [58]. Studies on transgender people who have not yet started HRT found that while overall brain volume and structure tend to align with their sex assigned at birth, the organization of their white matter — areas of the brain composed of insulated nerve fibers — more closely resembles their identified gender [59, 60]. Moreover, some brains of people with GI appear intermediate between the binary sexes, suggesting that brain differences are much more nuanced than previously thought [61]. Studies on transgender individuals and HRT have challenged the idea that male-female brain differences are immutable; brain structure is not fixed by biological sex but rather is sensitive to hormonal environments, aligning with the idea that individuals exhibit a mix of sex-associated traits rather than fitting into strict male or female categories [46, 49, 50].
HRT is widely recognized as the first line of medical treatment for GD in transgender individuals, significantly reducing psychological distress and improving quality of life [11, 13, 15]. Feminizing hormone therapy for male-to-female (MtF) transgender women typically involves estradiol, which can be administered via several routes, including oral/sublingual tablets, transdermal patches, or injections [63, 64]. Because estrogen alone may not fully suppress internal testosterone production in transgender women, an anti-androgen medication is usually prescribed in tandem to block androgen receptors and reduce testosterone production [64, 65, 66]. Cell receptors
function as tiny locks: when the appropriate molecule or ‘key’ is present, they bind together and trigger a cell response. Testosterone and estrogen bind to the same receptor, but testosterone takes priority when both are present [67]. Therefore, androgen blockers are implemented to prevent testosterone from binding, allowing estrogen to bind to the receptor and induce feminization [67]. Combining estradiol with effective testosterone suppression contributes to breast development, fat redistribution, skin softening, and other feminizing changes, typically over many months [68]. Physical changes not only improve gender congruence but also contribute to the mental health benefits seen in transgender women on HRT, such as reduced GD, anxiety, and depression [14]. Meanwhile, masculinizing therapy for female-tomale (FtM) transgender men involves the administration of testosterone to induce male characteristics [69]. Testosterone can be given as injections, gels, creams, patches, or newer long-acting formulations like implants [70]. Unlike MtF individuals, FtM hormone therapy does not necessitate an additional hormone blocker as testosterone is already prioritized over estrogen in binding to receptors [67, 71]. Overall, masculinizing HRT aids in relieving GD by facilitating changes such as a deepening of the voice, growth of facial and body hair, fat redistribution, a halting of the menstrual cycle, and clitoral enlargement [69].
For transgender youth, pubertal suppression is an early intervention that pauses the puberty of their biological sex until they are old enough for HRT [7, 72, 73]. Puberty blockers temporarily halt the signaling that drives puberty, which prevents unwanted development that can worsen dysphoria, such as breast growth or voice deepening [72, 74]. Clinically, puberty blockers have a long history of safe use in treating atypically early puberty and other pediatric hormonal conditions in cisgender youth [73]. In the context of GD, the use of puberty blockers has been endorsed by medical professionals for appropriately assessed youth [7, 11, 75]. The effects of puberty blockers are reversible — if the medication is stopped, puberty of the biological sex will resume [7, 17, 73]. By stalling puberty, transgender youth gain time to mature and consider their options. Because 96.5% to 98.1% of transgender youth who use hormone blockers will proceed to HRT later in life, hormone blockers allow them to do so without having undergone the unwanted, irreversible changes caused by puberty [7, 17, 76].Altogether, delaying puberty has been associated with better mental health, improved psychosocial functioning, and life satisfaction in transgender youth [15, 77, 78].
HRT does not just alter secondary sex characteristics — it also produces non-harmful changes in the brain [79]. The brain exhibits remarkable plasticity in response to hormonal changes, undergoing modifications that further support gender alignment and affirmation [49]. Transgender women on estrogen therapy undergo cortical thinning that makes their brain anatomy more similar to that of cisgender women, while transgender men on testosterone therapy show increases in gray matter volume that make their brains more like those of cisgender men [49, 51, 80]. The fact that HRT can reshape brain structures further underscores the brain’s adaptability, supporting the argument that changing hormone levels play a dynamic role in brain function across one's lifespan [81]. Changes in hormone levels have significant implications for transgender individuals undergoing medical transition, as HRT influences not just observable changes in physical appearance but also internal neural structures [81].
When transgender individuals undergo HRT, changes in sex hormones lead to measurable remodeling of brain tissue [49]. Transgender men taking high-dose testosterone have had increases in overall brain volume and growth of certain regions [50, 82]. Conversely, transgender women on estrogen with anti-androgen medication tend to experience volume decreases in some structures, such as the hippocampus [50]. A reduction in hippocampal volume is consistent with the feminization of the brain and may reflect a shift toward a female-typical structure [50, 58, 83]. Over time, HRT appears to be correlated with brain reorganization, a form of structural confirmation that parallels external bodily changes [50, 80]. It is important to note that many of these changes, such as variations in hippocampal or hypothalamic volume, are subtle
and not associated with impaired brain function [84]. No cognitive detriment has been identified from HRT; neither transgender men nor transgender women show any impairment in cognitive functioning compared to baseline [85]. Instead, alterations in brain structure likely reflect the brain adapting to function optimally under new hormonal conditions [49].
By alleviating GD and inducing physical changes congruent with one’s identity, HRT is strongly associated with improved mental health in transgender individuals [15]. Transgender individuals often experience chronic dysphoria and minority stress — how society’s mistreatment of stigmatized minority groups results in worse mental and physical health outcomes [86]. Chronic dysphoria and minority stress seem to contribute to the dysregulation of the hypothalamic-pituitary-adrenal axis, a hormonal system crucial in the role of fear and stress as well as sleep, leading to elevated levels of stress hormones and anxiety [87, 88, 89]. Transgender people who are denied gender-affirming care and are experiencing minority stress often exhibit high rates of depression, anxiety, and even potential cognitive detriments as a result of prolonged stress [89, 90]. The most crucial mental health outcome to consider is suicidality [91, 92, 93]. Transgender people experience disproportionately high rates of suicidal ideation and suicide attempts, largely due to internalized transphobia and societal stigma [91, 92, 93]. In their lifetime, 81.3% of transgender respondents to a recent survey had reported having suicidal ideation, with 42% having attempted suicide [91]. The heightened levels of psychological distress experienced by transgender people elucidate the necessity of early access to HRT as an important avenue for achieving mental stability and resilience [15, 89].
One of the most consistently documented outcomes of HRT is a significant reduction in depressive symptoms and anxiety [14]. In as little as three to six months, many individuals report relief from symptoms of depression and anxiety [13]. By 12 months on HRT, overall mood and general quality of life are improved in most patients [13]. Furthermore, access to hormone therapy is associated with a striking reduction in suicidal thoughts and behaviors [94]. A large study of U.S. transgender adults in 2022 found that those who received hormone therapy had a significantly lower incidence of recent suicidal ideation and past-year suicide attempts than those who desired but could not access HRT [89]. Moreover, individuals who had access to HRT during adolescence were less likely to experience suicidal ideation compared to those without access to HRT until adulthood [89]. Transgender people who started hormonal therapy as teens had fewer lifetime suicide attempts and lower incidence of substance abuse than those who started in their twenties or later [89]. Importantly, mental health improvements may be due not only to the psychological relief of gender affirmation but also to the hormonal effects on brain networks involved with self-perception [95, 96]. The message is clear: early and continued HRT saves lives [15, 89, 94].
Gender-affirming hormone therapy holds the potential to alleviate the fundamental discord between brain, body, and identity in some cases of GD by leveraging the brain’s plasticity and the transformative power of hormones [15, 38]. While HRT is not the only route by which transgender individuals can achieve inner peace, it is an evidence-based, life-saving treatment that must be accessible to those who desire it [15, 89, 94]. HRT demonstrates the intimate links between the brain, body, and mind, particularly regarding gender identity. By altering one’s internal hormone levels, HRT can initiate a positive feedback loop of well-being. Such well-being is displayed through improvement of mood and a reduction in suicidality, supporting HRT’s role as a medically necessary and profoundly effective treatment.
References on page 71
by Jack Matter | art by Lola Yost
An ordinary decision, such as what you order for lunch, could leave you vulnerable to parasitic tapeworms, unwanted guests that can wreak havoc on your body [1, 2, 3]. You may think the worstcase scenario from consuming undercooked or contaminated foods is a tapeworm in your gut, but they can actually invade your brain tissue as well [4]. Adult tapeworms use spiny hooks and suckers to latch on to the intestinal wall of a human host, siphoning nutrients from them [5]. As the worm grows, it sheds new eggs into the host’s feces. If another person ingests these eggs, the complex life cycle of the tapeworm begins to unfold [4]. The eggs can release tapeworm larvae that enter the host’s bloodstream and reach their most sensitive tissues, including the brain and spinal cord [4]. Neurocysticercosis (NCC) is a parasitic infection of the brain and spinal cord that begins when individuals accidentally ingest the eggs of tapeworms from the Taenia family, most often Taenia solium [1, 2, 6]. Some of the defining symptoms
of NCC include seizures, headaches, and increased pressure on the brain [3]. In fact, NCC is a leading cause of preventable epilepsy in many parts of the world [3]. Effectively addressing NCC in areas where it is common requires a holistic approach that integrates individually tailored treatment plans, improved diagnostic tools, and robust public health investments [3, 6].
On the outskirts of a farm, sewage runoff contaminated with tiny T. solium tapeworm eggs has seeped into the soil [3]. A pig sniffs around in the dirt, scavenging for food, and coincidentally ingests the eggs [3]. The eggs are the first stage of the tapeworm’s complex life cycle, which includes several larval phases before fully mature adult worms develop [7]. After the pig ingests the eggs that protect the tapeworm embryo, the larvae emerge from the egg to start the next phase of their life cycle in the pig’s intestines [3, 7]. The larvae burrow into the pig’s muscles and form protective fluid-filled sacs around themselves called cysticerci [3, 6]. Eventually, a farmer slaughters the cysticerci-infected pig and sells it to a food vendor. The vendor sears the pork kebab on a grill but fails to cook it all the way, leaving live larvae inside the cysticerci [8]. A traveler stopping for dinner buys the undercooked meat and unknowingly ingests the cysticerci containing tapeworm larvae [8]. Over the next several weeks, an adult tapeworm can emerge from each ingested cysticercus, mature in the traveler’s intestine, and produce more eggs, which are shed into the traveler’s feces [2].
The traveler continues on their journey and develops mild abdominal pain, unaware of their new guest [3]. They do not wash their hands thoroughly after using the restroom, and traces of tapeworm eggs are left on doorknobs, restaurant tables, and even directly on food shared with others in their hostel [3]. The hostel guests who come into contact with the shared surfaces ingest the eggs, unknowingly putting themselves at risk for a dangerous infection. Unlike the initial traveler who ingested cysticerci, a hostel guest named Paul ingests tapeworm eggs [4]. The larvae of
the tapeworm T. solium hatch from the eggs and invade Paul’s body [9]. The larvae then pass through Paul’s intestinal wall and enter the bloodstream, giving them access to muscle, the spinal cord, and brain tissues [2]. The larvae can embed in these tissues and develop into cysticerci, resulting in the infectious disease cysticercosis [2, 3, 10].
NCC, a specific type of cysticercosis, occurs when the hatched T. solium tapeworm larvae migrate through the blood to embed within the human brain or spinal cord, the organs that make up the central nervous system (CNS) [2]. The cells in the immune system can usually detect and respond to foreign threats like NCC in the body with the help of markers called antigens that are present on the outside of most cells [11]. Antigens trigger certain immune cells to produce corresponding molecules called antibodies. When antibodies bind to their specific antigens, they flag these foreign threats to activate immune responses that can ultimately kill an infection [11]. In the case of Paul’s parasitic infection, the immune system should react with an inflammatory response during which chemical signals are released to attract immune cells to the infection site [12]. Recruited immune cells would then release chemicals that can either destroy the parasite or contribute to further inflammation [12, 13]. Consequently, to successfully survive in the CNS, cysticerci must avoid being detected by the immune system [13].
While the immune system is designed to react swiftly to harmful invaders, cysticerci can suppress the host’s inflammatory responses, allowing them to go undetected by the immune system for extended periods of time [13, 14]. The absence of a robust inflammatory response may allow an individual with NCC to live completely symptom-free for many months [6, 13]. Cysticerci may use several strategies to avoid immune detection [13]. Say a bank robbery is underway; in this example, the robbers are the cysticerci, and the bank teller is an immune cell. The bank teller would normally spot the robbers and immediately alert authorities. In the case of a parasite, immune
cells would signal other immune cells to destroy the cysticerci. The robbers anticipate this, however, and create a diversion or tie up the teller to prevent the alarm from ever going off. Similarly, cysticerci release antigens that draw immune cells away from them or prevent immune signaling cells from alerting the immune killer cells. As a result, the immune system remains unaware of the threat. Another strategy the bank robbers may use is a disguise, like dressing up as bank employees. The cysticerci can mask themselves from the immune system by covering their outer layer with the host’s own antibodies, tricking immune cells into thinking that the cysticerci are a part of the body and belong there [13]. Using these strategies, cysticerci can persist inside a host for a long time without causing any symptoms [6, 13].
After months or even years, cysticerci can break down for unknown reasons, losing their ability to evade the immune system and subsequently activating an inflammatory response from the host [6, 9, 13]. Paul’s more serious symptoms, such as intense headaches and seizures, started with the activation of the inflammatory response [3, 9, 14]. For many individuals, the severity of these symptoms is typically associated with the intensity of the immune response in the brain [4, 9]. Following the immune system response, the cells that comprise the blood-brain barrier — a protective layer of cells that selectively prevent substances from entering the brain — also experience disruptions to their function [9, 13]. When the bloodbrain barrier is compromised, immune cells usually excluded from the brain are allowed in and can then identify and attack broken-down cysticerci directly [9, 13]. Furthermore, immune attacks on cysticerci can be both beneficial and harmful [13]. On one hand, the cysticerci are being destroyed and the larvae are being killed, removing live parasites from the body. On the other hand, the destruction of cysticerci can lead to dangerous levels of immune inflammation in the brain that can severely damage surrounding tissues and cause more extreme symptoms [13].
A variety of NCC symptoms can arise depending on where the cysticerci are physically located within the CNS [6]. Broadly, there are two main types of NCC in the brain [10]. The most common type of NCC occurs when cysticerci form in the brain tissue itself — made up of nerves and supportive cells — called parenchymal NCC. Another type of NCC develops when cysticerci form in the spaces between brain tissues, including fluid-filled spaces and blood vessels, known as extraparenchymal NCC. Each type of NCC affects different brain regions and causes varying symptoms [10]. In parenchymal NCC, physical pressure or vessel blockages from the cysticerci and inflammation from the immune response may damage brain tissue, causing symptoms including headaches, vomiting, and even numbness in specific areas like the face [10, 15]. Paul, our hostel guest, suffers from the less common type, extraparenchymal NCC, which can cause similar symptoms to parenchymal NCC but may also lead to other fatal complications, such as stroke or increased pressure within the skull [15, 16]. Increased skull pressure is particularly dangerous because it can squeeze the brain and its blood vessels, ultimately leading to tissue damage, which can be permanent and even deadly [17].
Both types of NCC can also trigger seizures and lead to the development of epilepsy, an umbrella term for a disorder in which brain abnormalities cause recurring seizures [10, 18]. NCC is a leading cause of epilepsy in areas of the world where the parasitic infection is most prevalent [18]. The exact reason why NCC causes seizures is poorly understood, but it is believed that the inflammatory response plays a significant role [19]. Additionally, the likelihood of developing epilepsy from infectious and other types of lesions — areas of damaged tissue — depends on their location and size in certain parts of the brain [10, 20, 21]. Cysticerci are considered a type of lesion that contributes to scarring and damage to surrounding nerve cells, which may lead to epilepsy, as seen in Paul’s case [10]. Repeated seizures can continue even after the cysticerci are destroyed and become hardened, inactive lesions called calcified cysts [10, 19]. As many as half of individuals with NCC-associated epilepsy and calcified cysts experience chronic seizures ranging from months to years after cysticerci become calcified, further complicating our understanding of how cysticerci contribute to epilepsy. One possible explanation is that brain tissue may swell and scar around these calcified cysts, which is often noticed in individuals with seizures [10, 19]. However, it is unclear if the swelling and scarring cause the seizures or if seizures lead to these changes [19]. Another idea
is that the calcified cysts might slowly release large amounts of calcium into the brain. Calcium is an important chemical messenger in many normal signaling processes, but too much calcium can harm brain cells. If nearby brain cells sustain damage or begin to die from excessive calcium exposure, they could release increased amounts of other chemical signaling molecules associated with seizures. Surgical removal of calcified cysts decreased the occurrence of seizures in a limited number of individuals, but the risk of brain surgery may not outweigh the theoretical benefit. Overall, the identification and elimination of cysticerci before they degenerate and harden into calcified cysts significantly lowers the risk of developing chronic epilepsy. The range of symptoms caused by NCC, including seizures and epilepsy, extends beyond those experienced from parenchymal and extraparenchymal NCC [19].
Beyond the brain, NCC can also occur and trigger symptoms in other parts of the CNS, such as the spinal cord [15]. Instances of NCC originating in the spine are rare but nonetheless possible [22]. Affected individuals may experience different symptoms depending on where cysticerci are located along the spinal cord [15, 23]. The presence of cysticerci can lead to compression of the spinal cord, which may cause symptoms including muscle weakness and movement problems [23]. In some cases, cysticerci may develop across several parts of the spine, leading to widespread dysfunction [23]. Dysfunction can include anything from general feelings of weakness to total paralysis of the limbs [23, 24]. The vast range of symptoms and respective classifications make NCC a complex disease that can affect many different aspects of a person’s health, contributing to both diagnostic and treatment challenges [15].
Diagnosing any kind of NCC typically requires a combination of screening, medical imaging, and blood tests [6, 25]. If Paul comes into a clinic with possible symptoms of NCC, healthcare professionals will first look at Paul’s medical history and risk factors for clues — such as whether he lives around livestock — that could increase his risk of becoming infected [6, 25]. Healthcare providers may then image his brain using techniques such as magnetic resonance imaging (MRI), which utilizes powerful magnets and radio waves to generate detailed pictures of the brain [6, 25, 26]. If cysticerci are seen or suspected in an MRI or other types of diagnostic images, follow-up blood
tests can use antibodies to detect antigens associated with the T. solium tapeworm [27, 28, 29]. A positive blood test for these antigens helps providers confirm Paul’s NCC diagnosis [27, 28, 29]. Following NCC diagnosis, Paul’s treatment plan would depend on where the cysticerci are located, how many there are, and their stage of degeneration [6, 31, 32]. A ‘one size fits all’ treatment plan is therefore ineffective for NCC, but the same general strategies are followed in most cases [6, 31, 32]. First, a healthcare provider might focus on reducing Paul’s symptoms by using several different medications [30, 32]. Some common examples include corticosteroids to lower inflammation and swelling and antiepileptics to reduce the frequency and intensity of seizures [30, 32]. When combined, these medications can target multiple symptoms at once, which can improve overall treatment outcomes [30, 32]. Alongside symptom relief, a provider may simultaneously prescribe drugs that kill Paul’s cysticerci, known as cysticidal drugs [4, 30]. Cysticidal treatments work in a variety of ways — most commonly by slowly interfering with the vital functions of cysticerci until they break apart [30]. Once cysticerci begin to die from cysticidal treatment, their contents, including antigens, leak out and can either generate or intensify a preexisting inflammatory response [30]. Concurrent use of corticosteroids alongside cysticidal drugs can help ease any excessive inflammation [2, 15].
UNDERCOOKED AND OVERLOOKED
Still, cysticidal drugs are not a viable option for every person, even when combined with anti-inflammatory corticosteroids [30]. Symptoms might not improve at first and could initially worsen for some individuals [30]. Worsening symptoms are especially dangerous in people who already have severe symptoms caused by NCC, such as increased pressure in the skull [30]. Paul has an acute case with a large cysticercus that is causing dangerous, rapidly appearing symptoms that led him to go to the emergency room [1, 33]. The large size of his cysticercus may mean it could take days to shrink using drugs and cause complications from inflammation [33]. With such a severe case, Paul might not survive long enough for this treatment to run its course, and a medical team at a hospital would likely decide that surgical removal is the best option [33]. Because of differing factors and treatments, NCC can be complicated to manage but is considered curable [34]. Still, the disease outlook following treatment depends on the individual case, especially cysticerci location [34].
Early detection and diagnosis of NCC are particularly challenging in regions where the disease is most common [35]. NCC prevalence is highest in certain parts of Africa, Asia, and South America, typically in countries that lack critical infrastructure [35]. Inadequate sanitation and health education systems are just some examples of weak infrastructure that public health authorities can struggle with [3, 25, 36]. As with many treatable diseases, a shortage of resources can mean many people do not get the proper testing or treatment they need [37]. Likewise, NCC is most likely underestimated and misdiagnosed because the symptoms, such as headaches and seizures, can look very similar to those of other brain disorders [25, 36]. In addition, carriers of the T. solium parasite that could pass eggs on to other people may be asymptomatic, so they are seldom screened [36]. When combined with a general lack of public awareness surrounding NCC, limited screening, and more misdiagnoses contribute to the challenges surrounding the disease [36].
NCC remains a neglected and likely underreported disease, lacking adequate monitoring in many of the countries most deeply impacted [2, 10, 35]. As a result, while increased rates of epilepsy are likely a consequence of increased rates of NCC, large datasets on NCC occurrence can be lacking [2, 10]. Mandatory case reporting could help health authorities more accurately assess how NCC affects
communities, both socially and economically [2]. Overall, addressing challenges related to NCC would benefit from a multifaceted approach. Improving sanitation infrastructure — such as increased access to clean water, better waste management, and campaigns to deworm livestock — alongside public education on food safety and disease awareness could significantly reduce the burden of NCC [10]. In addition to enhanced monitoring and infrastructure, prevention strategies are particularly essential. Improving access to rapid diagnostic blood tests that do not require a lab, human vaccine development, and enhanced biological data collection would make a large impact on the field [3]. Investment in affordable, easy-to-use diagnostic tools like immediate-use blood testing kits could improve early detection, particularly in settings with limited resources [3]. Currently, one of the most definitive ways to confirm an infection is by visualizing the parasite in a tissue sample or by MRI [38]. However, this is impractical for widespread use due to the financial burden of specialized equipment and staff [3, 38]. Moreover, blood tests that can detect T. solium in a controlled hospital setting are not sensitive or specific enough to be used widely in the field and are relatively expensive [3]. Research into making blood tests cheaper and more precise would greatly improve disease control efforts [3].
Furthermore, while a vaccine is available for pig cysticercosis, no such vaccine exists for humans [3]. The lack of a human vaccine is partly because developing vaccines for pigs is cheaper, and vaccinating humans has not been considered practical in the past because not enough highly specific target antigens have been discovered for T. solium [3]. A theoretical vaccine would rely on stimulating the immune system by introducing select, very specific antigens associated with T. solium so that the immune system could remember and recognize the actual tapeworm in the case of future exposure [39]. When introduced to these select T. solium antigens, the immune system produces antibodies that match and bind to them [39]. In an active parasitic infection, these antibodies would then be able to neutralize a parasite’s ability to establish in the body [39]. Progress on a human vaccine is slow, but some new specific vaccine targets have recently been discovered, including a protein associated with T. solium reproductive cycles [3, 40]. Adding a T. solium vaccine to childhood vaccination plans could considerably reduce infections in communities where the parasite is most often found [3]. Still, any potential vaccine would face challenges due to cost; even effective vaccines designed for pigs
have not been widely used due to financial barriers [3]. Reducing the prevalence of NCC and associated health issues requires further long-term investment [3, 35, 41].
NCC is a significant global public health challenge at the intersection of complex biological and socioeconomic considerations [3]. The complexity of NCC is demonstrated by individual differences in how NCC manifests and unanswered questions about how the disease drives symptoms from mild headaches to fatal neurological complications [1, 6, 30]. Longterm work addressing NCC will require a comprehensive approach that brings clinical practice and policy together [35, 41]. Global financial investment in improved infrastructure, public education, and clinical tools is required [41]. By combining targeted therapies, improved early detection, and preventive strategies, we can work toward reducing and someday eliminating the global impact of NCC and protect communities from an entirely preventable cause of serious neurological symptoms [3].
References on page 76
by Lucia Holbrook-Brown | art by Ansen Chamberlain
In the second century CE, the Greek philosopher Aelius Aristides arrived in Rome, motivated by his early career success [1]. However, Aristides’ ambitions were stalled when he fell ill and became consumed by his health [2]. Aristides suffered debilitating symptoms, including chronically severe abdominal pain and persistent fatigue [1]. His writings reveal disproportionate anxiety related to his health, which exacerbated his disabilities [1, 2]. Aristides turned to Asclepius, the preeminent healing god, seeking comfort, guidance, and healing through ritual and devotion [2]. Though Aristides experienced occasional remissions, his illness never truly resolved, and he died decades later, still in pursuit of a cure [2]. Aristides’s story, though ancient, is not unfamiliar to our age. Today, similar chronic diseases continue to burden not only individuals and their families but also clinicians and healthcare systems. Disorders like the one Aristides suffered from — now referred to as functional somatic disorders (FSDs) — account for 30% of primary care visits and $174 billion annually. Despite the millennia between Aristides and ourselves, many are trapped in cycles of persistent pain, left only with costly medical consultations, heightened health anxiety, and limited effective treatments [3].
FSDs refer to a group of disorders characterized by chronic, distressing body symptoms — such as pain, fatigue, and gastrointestinal issues — that are not fully accounted for by identifiable medical conditions [4, 5]. These physical symptoms, called somatic symptoms, significantly disrupt daily life despite an absence of observable disease [6]. The leading theoretical model contends that somatic symptoms arise from psychological factors [7, 8]. While fleeting somatic symptoms are common, such as experiencing
butterflies before public speaking, FSDs are diagnosed when physical symptoms are chronic, impairing, and disproportionate to any identifiable medical cause [9, 10]. The classification of FSDs remains highly contested [11]. The Diagnostic and Statistical Manual of Mental Disorders (DSM-5-TR) categorizes FSDs under Somatic Symptoms and Related Disorders, focusing on the disproportionate psychological distress associated with somatic complaints, regardless of whether a medical cause exists [12, 13]. Similarly,
the International Classification of Diseases (ICD-11) outlines bodily distress syndrome, which is characterized by excessive concern with bodily symptoms, often but not exclusively due to an unattributable cause [14, 15]. Notably, the ICD-11 framework also includes chronic widespread pain (CWP), which explicitly requires the exclusion of an identifiable medical cause [14]. CWP recognizes psychological factors, but it does not rely on labeling an individual’s concern as excessive [16]. Both the ICD and DSM overemphasize psychological aspects of disease and fail to provide classifications that integrate mind and body [17].
The limited understanding of FSDs’ underlying mechanisms has resulted in uncertainty surrounding their classification [11]. The diagnostic approach relies on ruling out abnormal biological processes that may explain unusual somatic symptoms, rather than directly looking for markers that identify FSD [4, 17]. As a result, some experts do not view classification of FSDs into individual syndromes as a current priority because they believe the underlying biological mechanisms must be discovered before classification can accurately proceed [7]. However, other experts believe that regardless of the unknown mechanism, FSDs should be classified with chronic illnesses [7]. To address the controversy in classification, the term functional somatic disorder was proposed in anticipation of the 2022 revision of the ICD [17]. Though the term functional somatic disorder is not universally accepted, it emphasizes an integrated approach of the mind and body that is missing from past definitions [17].
FSDs encompass a range of conditions — such as functional neurological disorder (FND), irritable bowel syndrome (IBS), and fibromyalgia — that share a common feature: persistent, distressing physical symptoms that lack an identifiable biomedical cause [18, 19, 20, 21]. FND presents with altered sensory or motor function — including impaired movement, chronic dizziness, brain fog, and non-epileptic seizures — but is only diagnosed in the absence of a detectable neurological condition [19, 22, 23]. Non-epileptic seizures, for instance, may resemble epileptic convulsions — presenting with full-body convulsions, twitching limbs, lapses in awareness, and muscle weakness or paralysis — but occur without measurable abnormal brain electrical activity [24, 25, 26, 27]. Management of FND symptoms is expensive and often futile, as seen with non-epileptic seizures, which do not respond to anti-seizure medications [22, 28, 29]. IBS involves chronic abdominal pain and disordered bowel habits [30]. IBS is widespread, affecting an estimated 12% of the global population; however, treatment is largely ineffective, leaving almost one billion people with unmanaged symptoms [20, 30]. Fibromyalgia is a chronic musculoskeletal pain disorder characterized by widespread muscle aches, fatigue, and sleep disturbances [21, 31]. The intense pain and fatigue associated with fibromyalgia can significantly decrease a person's quality of life, which has been hypothesized to increase suicide risk [31, 32, 33]. Despite conflicting views in classification, the categorization of specific FSDs into disorders such as IBS remains useful for treating symptoms restricted to one organ or body system [17].
In order to find a clearer basis for diagnosing and defining FSD, a multifaceted approach is required, which integrates medical and psychological perspectives in pursuit of identifying biomarkers: biological indicators of disease [6, 19, 34]. Biomarkers measure results of biological processes, such as proteins or genes, that reflect physiological processes [35]. Improved clinician understanding of disorders can be facilitated with biomarkers, as they can aid diagnostic accuracy, treatment, and disease trajectory [34, 36, 37]. Dysfunction within and between the immune system and the brain is being explored as a potential contributor to FSD and could thus contribute to diagnostic criteria [17, 38, 39, 40]. In FSDs, the immune system is activated by systemic low-grade inflammation (SLI), a state in which tissues express
inflammation without resulting in structural or functional change [41, 42]. SLI is caused by cytokines, small proteins produced by immune cells that relay messages between cells to promote cell functions responsible for bodily inflammation and may contribute to the somatic symptoms seen in FSDs [41, 42]. Two specific cytokines are biomarkers of SLI in FSD: IL-6 and hs-CRP [42]. IL-6 induces the expression of hs-CRP, which mediates inflammation. IL-6 and hs-CRP are important indicators of inflammation and are considered potential biomarkers for FSD that could help uncover the mechanism. SLI associated with FSDs is characterized by elevated IL-6 and hs-CRP levels in the blood, a marker that is often unique to FSDs [42]. High levels of IL-6 and hsCRP are also associated with the promotion of inflammation, increasing physical symptom intensity and frequency, and leading to heightened pain sensitivity [43]. However, IL-6 and hsCRP are not officially recognized as diagnostic tools in clinical practice [42]. Understanding the role of the immune system in FSD allows scientists to develop more precise diagnostics and targeted therapies, leading to better outcomes for people with FSD [36]. Therapies might target the immune pathways involved, should they be elucidated [36].
Abnormalities in the brain are being investigated in an attempt to uncover the cause of FSDs, with the existing research focusing primarily on functional neurological disorder [44, 45, 46]. Neuroimaging has uncovered differences in brain structure and function in individuals with FND compared to individuals without FND [28]. Individuals who reported severe impairment in daily functioning due to FND displayed reduced volume of their insular cortex, a brain region that influences one’s sense of self-awareness, emotional processing, and interoception [28, 47]. Interoception refers to the awareness of the internal bodily signals, such as the sensation of pain [48]. When
interoception is altered, individuals may experience increased pain, leading to more intense symptoms [28, 49, 50]. Functional imaging is an additional tool in the characterization of FND, as it can provide evidence of activity changes in specific areas of the brain [28]. In individuals with FND, there can be decreased activity in areas associated with movement, such as the supplementary motor area (SMA), and increased activity in areas in the insular cortex [28, 51]. Additionally, those with FND have increased neuronal activity between parts of the limbic system, a part of the brain that regulates emotions, and the motor circuit [28]. The increased activity potentially indicates that there is heightened limbic influence over motor behavior [28]. Promoted limbic and insular activity may shed light on how psychological symptoms lead to the manifestation of seizures and other somatic symptoms in FND [28, 51, 52]. Although brain activity changes have only been concretely studied in FND, similar mechanisms may be at play in other FSDs, warranting further research [53].
In addition to the immune system and brain involvement in symptom development, FSDs are influenced by an individual's psychological health [54]. FND, fibromyalgia, and IBS often occur simultaneously with other diseases, referred to as comorbidities [54]. Anxiety and depression are common comorbidities, impacting about one-third of those with IBS and half of those with either fibromyalgia or FND [55, 56, 57, 58]. In fibromyalgia and IBS, comorbid mental disorders can exacerbate somatic symptoms [59, 60]. However, the presence of a comorbid disorder with FND does not necessarily exacerbate neurological symptoms such as non-epileptic seizures [58]. Understanding the complex interactions between psychological comorbidities and FSDs is essential for refining diagnosis and treatment to improve outcomes for those affected [23, 61].
Treatment for FSDs requires a multifaceted approach, involving psychiatrists, general practitioners, and medical specialists [62]. A large focus of treatment for FSDs is improving psychological health through the use of psychotherapies, such as cognitive behavioral therapy (CBT) and psychodynamic therapy (PDT) [63]. CBT posits that thoughts and beliefs can promote and maintain mental disorders, and therapy aims to unlearn these [64]. In the context of FND, CBT aims to reduce symptoms by increasing one’s bodily awareness, challenging symptom-related anxieties, and changing illness-promoting behaviors [65]. PDT is based on practices that focus on the individual’s internal world and aims to deconstruct misperceptions and misinterpretations of external realities by making the unconscious conscious [66]. PDT for FND aims to link symptoms to an individual’s emotional experiences and thus regulate somatic symptoms by regulating emotions [65]. Non-pharmaceutical therapies such as CBT show promise for treating fibromyalgia, but their precise effects on symptom management are still being investigated [67, 68]. Furthermore, a lack of long-term research on CBT’s ability to manage IBS symptoms presents uncertainty around the use of psychotherapy as a treatment option [62]. While CBT and PDT have been able to relieve some symptoms, they do not cure people with FSD [65].
Psychiatric pharmaceutical intervention can supplement psychological therapies in the treatment of FSDs [36]. Often, people with FSDs are prescribed antidepressants like selective serotonin reuptake inhibitors (SSRIs) or serotonin-norepinephrine reuptake Inhibitors (SNRIs), which increase the levels of the chemical messengers serotonin and norepinephrine
in the brain [36, 69]. Increased levels of serotonin and norepinephrine can help stabilize mood and potentially limit psychological triggers to somatic symptoms [70]. Tricyclic antidepressants work similarly to SNRIs by targeting both serotonin and norepinephrine. However, unlike SNRIs, tricyclic antidepressants target a broader range of neurotransmitters, often leading to more severe side effects [37, 71]. When prescribed for FSDs, tricyclic antidepressants are often slightly more effective, but have more severe side effects [37, 71]. Despite the common use of SSRIs, SNRIs, and tricyclic antidepressants in the treatment of FSDs, their efficacy varies [72, 73]. Antipsychotics are other pharmaceutical interventions used to treat FDSs that regulate dopamine, a neurotransmitter involved in reward processing and motivation, and serotonin [72, 74]. However, antipsychotics have shown limited success in the treatment of FSDs [72, 75, 76]. Psychiatric pharmaceutical interventions often fail, leading to increased frustration among doctors and people with FSDs [36]. Fibromyalgia, IBS, and FND are chronic conditions, and as a result, psychological health interventions alone are often not enough, and additional treatments are required [63].
Another area of focus for the treatment of FSDs is improving physical health [36]. A common first-line treatment for FSDs is physical therapy [36]. Individuals with FSD often experience physical disability, leading to a more sedentary lifestyle that contributes to poorer physical health and intensifies existing symptoms [77]. Physical therapy that is especially focused on mobility and strength building can reduce the pain associated with fibromyalgia and IBS, as well as the risk of developing comorbid disorders [77, 78]. While physical therapy has not been studied in the management of FND, its success in treating fibromyalgia and IBS suggests that it could be beneficial in treating FND [79]. Another common treatment for FSDs is neurostimulation, which uses electrical stimulation to modulate irregular brain activity [80]. Neurostimulation has varying degrees of invasiveness and works by stimulating underactive areas, suppressing overactive areas, and disrupting abnormal activity in the brain [81, 82, 80]. Certain neurostimulation is believed to reduce inflammation throughout the body, which may help alleviate the pain symptoms associated with FSDs [83]. With exceptional promise in treating IBS, neurostimulation has demonstrated both a reduction in abdominal pain and regulation of bowel movements [84]. Although treatments are progressing, there has yet to be a broadly successful treatment for FSDs [36].
From Aristides’s time to today, chronic unexplained physical symptoms have imposed a burden on individuals and their healthcare systems. FSDs, especially FND, IBS, and fibromyalgia, remain challenging to classify, diagnose, and treat due to the absence of identifiable biomarkers, contested diagnostic criteria, and uncertain origins [4, 11, 19]. Although progress has been made in the recognition of FSDs as complex psychological and physiological disorders, a definitive understanding of FSDs remains elusive [8]. Emerging research on inflammatory biomarkers, neuroimaging findings, and psychological involvement offers promising directions to guide the field but has yet to yield a conclusive understanding of FSD mechanisms [28, 42, 54]. While current FSD treatment, including psychotherapy, medication, physical therapy, and neurostimulation, can offer symptom relief, there is no cure [36, 65]. Ultimately, the path forward lies in uncovering the underlying causes of FSDs, investing in multidisciplinary research, and developing evidence-based treatment strategies.
References on page 78
by Kaitlin Raskin | art by Mischa Landgarten & Iris Li
Millions of years of evolution have led chili pepper plants from Central and South America to possess a clever pain-inducing mechanism that deters pesky animals from eating them [1]. However, one species simply hasn’t gotten the hint: humans. They chewed on the fruit of the chili pepper plant and, despite feeling pain, they enjoyed it. Humans have since harnessed the plant’s active spicy ingredient, capsaicin, for culinary and medicinal purposes. Capsaicin naturally occurs in plants in the genus Capsicum, playing an important role in seed germination and protection from parasites since aversion to consuming capsaicin typically deters animals from eating Capsicum plants [1]. Besides its notable utility in the culinary world, capsaicin is also found in self-defense products such as pepper spray and has been traditionally used in medicine to relieve coughs and sore throats [2]. The interaction between capsaicin and the nervous system not only influences physiological responses to spicy food but also offers potential for pain relief [3, 4]. Hence, capsaicin is a promising alternative for treating chronic pain conditions, especially given the limited effectiveness and adverse side effects of many current pain medications [4, 5, 6].
When we eat spicy food, capsaicin molecules bind to and activate cell receptors responsible for receiving and processing pain signals [7]. Specifically, capsaicin activates transient receptor potential vanilloid 1 (TRPV1) receptors, which are found in nociceptive, or pain-sensing, neurons located in areas like the mouth, tongue, and skin [7, 8]. TRPV1 receptors are also activated by hot foods and beverages, linking the experiences of eating hot and spicy food [7]. When capsaicin binds to TRPV1 receptors, the molecule causes nociceptive neurons to fire, triggering the release of substance P and other pain-related signaling molecules from sensory nerve endings [9]. The signaling molecules travel through the body, relaying the pain signal to other neurons and eventually reaching the brain [1].
Through the pain-signaling cascade, capsaicin stimulates the autonomic nervous system, which is constantly regulating essential bodily functions, such as heart rate and breathing [10, 11]. Capsaicin triggers the ‘fight or flight’ response from the sympathetic nervous system (SNS), a branch of the autonomic nervous system that helps the body respond to stressful stimuli [11]. When eating a seemingly harmless taco on Taco Tuesday, a jalapeño may activate your SNS,
leading to increased heart rate, perspiration, and a burning pain sensation in your mouth [12]. Since capsaicin and hot food activate heat and pain-sensing TRPV1 receptors, both stimuli can trick the nervous system into thinking the body is overheating [13]. Like putting out a fire, the brain relays signals to the body to activate bodily cooling mechanisms [10, 14, 15]. Autonomic responses like increased sweating, salivation, and blood flow can occur as a result of the jalapeño [10, 14, 15]. Physiological responses to the pain-signaling cascade may consequently lead you to try to reduce the pain and alleviate these symptoms [10, 14].
Picture yourself eagerly preparing a fiery bowl of spicy Buldak Ramen for dinner. You take a bite and are immediately hit with a wave of pain as you begin to sweat, and your mouth waters ferociously — what do you do next? Your first instinct is to gulp down the glass of water next to you to alleviate the pain. Unfortunately, water doesn’t work because capsaicin is a hydrophobic substance, meaning it does not dissolve in water [16]. Pivoting to plan B, you frantically pour and drink a glass of milk, experiencing relief as the heat dissipates. Milk contains relatively hydrophobic casein molecules that surround and isolate the hydrophobic capsaicin molecules from the TRPV1 receptors [16, 17]. Similar to how soap washes away grease, the casein molecules effectively wash away the capsaicin, alleviating the capsaicin-induced burning sensation [16, 18].
If spicy food causes pain, why would someone ever choose to eat Flamin’ Hot Cheetos instead of regular Cheetos? After spicy food activates nociceptive neurons and causes a pain response, the body tries to counteract the pain signals by releasing pain-relieving molecules called endorphins [19]. Endorphins are hormones that are secreted by the pituitary gland, an area of the brain responsible for producing hormones that indirectly regulate many bodily functions such as growth and metabolism [20, 21]. Endorphins bind to opioid receptors, which are also the targets for pain-relieving opioid drugs like morphine [20]. When endorphins bind to opioid receptors, they prevent the release of pain-signaling molecules like substance P, reducing the sensation of pain [19, 22, 23]. In the brain and spinal cord, endorphins also stimulate an increase in dopamine, a chemical messenger transmitted between cells in the brain’s reward system [19]. The feeling of reward associated with dopamine release is a central motivating factor in our consumption of spicy food [19, 24]. Through the release of endorphins and dopamine, spicy food contributes to feelings of euphoria and leaves us wanting more, which may explain why some people choose to eat Flamin’ Hot Cheetos despite the subsequent pain responses [24].
On an individual level, personality traits also influence whether or not someone enjoys eating spicy food [25, 26]. Imagine you are at an amusement park, deciding between riding the roller coaster or the carousel. You choose the carousel, preferring a familiar and gentle experience over the excitement and unpredictability of the roller coaster. Consistently choosing the carousel over the rollercoaster can reflect a dislike of unfamiliar experiences. Similarly, individuals who don’t like spicy food are often more reluctant to try unfamiliar dishes [27]. People who don’t like spicy food are also more likely to experience disgust, responding more negatively to offensive stimuli such as rotten food and bodily fluids [27]. Those who dislike spicy food also tend to exhibit heightened reactions to negative consequences and are less motivated to seek out novel and complex experiences that produce feelings of reward [27, 28]. Now, imagine you had instead chosen to ride the roller coaster, finding thrill in unexpected acceleration changes and gravity-defying plunges. Individuals with greater sensitivity to feelings of reward are also generally more likely to enjoy eating spicy food [25, 26, 29]. Just as personality influences our choice of amusement park rides,
it also plays a significant role in our food preferences, with risk-taking and sensitivity to reward often driving the enjoyment of spicy foods [26, 29]. But even if you tend to prefer the carousel and avoid taking risks, there is still hope for developing a tolerance and finding enjoyment in spicy food [30].
Repeated consumption of spicy foods can lead to desensitization, a process in which the painful sensations triggered by capsaicin diminish [30]. Suppose you decide to challenge yourself by ordering Buffalo Wild Wings with Mango Habanero sauce every day for two weeks. For the first few days of the challenge, the heat from the sauce is unbearable, and your mouth is on fire. However, the spice level begins to feel tolerable over the course of the two-week period, and you start to fully appreciate the sweetness and complex flavors of the spicy sauce [1, 2, 30]. You experience a reduced pain response because consuming capsaicin at high doses or with prolonged exposure can lead to desensitization of the TRPV1 receptor pathway [1, 2, 30]. Constant activation of TRPV1 receptors by capsaicin or painful heat desensitizes the receptors for extended periods of time, preventing nociceptive neurons from firing [2]. As a result, there is a decrease in responses like sweating and salivation to a variety of TRPV1-related pain stimuli [1, 2, 30]. Additionally, when capsaicin repeatedly binds to TRPV1 receptors, an abundance of substance P pain-signaling molecules are released from nociceptive neurons [1, 2]. Once the supply of substance P becomes exhausted, the nociceptive neurons become functionally silent
as they can no longer communicate pain signals to the brain [1, 2]. Preventing the spread of the pain-signaling cascade contributes to TRPV1 desensitization [1, 2]. After being successfully desensitized to the spiciness of Mango Habanero sauce, you then take a year-long hiatus from eating spicy wings before ordering some more. You’re certain that you will be able to handle the spice as you did before. However, upon consumption, you are shocked to find that, yet again, your mouth is burning, and your eyes are watering. Unfortunately, at low doses of capsaicin, desensitization must be maintained by eating spicy food on a somewhat regular basis [30]. Although capsaicin works to silence nociceptive neurons, explaining the initial desensitization, the molecule simultaneously triggers the growth and regeneration of these neurons through a different signaling pathway [30, 31, 32]. Capsaicin binds to TRPV1 receptors, causing calcium ions to rush into the neurons and initiate a signaling cascade that activates a protein involved in growth and repair [32]. Once the neurons are repaired after several weeks or months, they may return to transmitting pain signals through the spinal cord and to the brain, causing a return of sensitivity to the Mango Habanero sauce [31, 32].
Capsaicin’s ability to induce desensitization by impairing TRPV1 receptors and silencing nociceptive neurons allows the molecule to be used pharmacologically to treat various pain conditions [1, 2]. TRPV1 receptors are not only located in areas that come into contact with food, such as the mouth, but also throughout the body as part of the peripheral nervous system, which allows neural signals to be sent between the rest of the body and the brain and spinal cord [33]. Thus, the pain-relieving properties of capsaicin can extend beyond the realm of culinary experiences and can be effectively utilized in clinical settings [32]. Capsaicin treatment can be used to facilitate pain relief for those suffering from neuropathic pain [2]. Neuropathic pain refers to pain stemming from nerve damage, which arises from disorders affecting sensory nerves [32, 34]. Affecting roughly 7-10% of the global population, neuropathic pain disorders include diabetic neuropathy as well as chronic pain and phantom limb pain (PLP) [2, 32]. Neuropathic pain for people with diabetic neuropathy, in which elevated blood sugar levels lead to nerve damage, often takes the form of a persistent pinching feeling in their legs and feet [35]. Moreover, individuals with amputated limbs often report experiencing PLP, which refers to an unexplainable stabbing or tingling sensation that seems to originate from the missing limb [36, 37, 38]. Neuropathic pain disorders are
often difficult to treat due to the variability underlying nerve damage and dysfunction [39]. However, capsaicin provides a unique approach to complex diagnoses by inducing pain desensitization [3, 4].
Unlike pre-existing treatments for neuropathic pain disorders, capsaicin offers an alternative avenue for pain management through the skin [2, 32]. Capsaicin is commonly used as an ingredient in topical formulations, meaning it is applied directly to the skin with a patch or as a cream [2, 32]. Though capsaicin application to the skin initially increases pain sensitivity through TRPV1 receptor activation, high concentrations or repeated applications of topical capsaicin can lead to persistent pain desensitization [33]. As a result, pain signals are prevented from reaching the brain, alleviating pain over time [2, 3, 34]. Medications utilizing capsaicin are unique in that they work through the peripheral nervous system, more directly targeting nerves in an affected area [40, 41]. In contrast, many long-standing medications for disorders like diabetic neuropathy, such as antidepressants and anticonvulsants, act through the central nervous system to interfere with pain processing in the brain and spinal cord [40, 41, 42, 43].
Since antidepressants and anticonvulsants don’t treat one specific area of pain, multiple neurotransmitter systems are affected, which can contribute to side effects [44]. While antidepressants and anticonvulsants have been traditionally used to treat depression and seizures, they’ve also been established as effective first-line treatments for diabetic neuropathy [44, 45]. However, these medications are associated with a myriad of adverse side effects, including dry mouth, constipation, dizziness, and headaches [44, 45]. Opioids, which may be administered when firstline treatments are ineffective in reducing pain, are also risky, potentially leading to addiction and abuse [32]. In comparison, topical capsaicin therapies provide an alternative form of treatment that circumvents many potential risks and side effects [45]. When a high concentration of topical capsaicin formula was applied to the feet of people with diabetic neuropathy, the pain was significantly reduced over two months [45, 46]. However, capsaicin administration was still associated with a burning sensation and pain at the application site despite the application of a local anesthetic before treatment [34, 44, 45, 46]. Fortunately, this pain dissipates over time following the initial administration. The pain relief from a high concentration of topical capsaicin is long-lasting, persisting up to three months after one application [44, 47]. Relative to an anticonvulsant, topical
capsaicin treatments provide pain relief with a faster onset of action, greater patient satisfaction, and fewer side effects [46]. As a result, in 2020, the Food and Drug Administration approved topical capsaicin as a treatment for diabetic neuropathy [45]. Like diabetic neuropathy treatments, antidepressant and anticonvulsant treatments for chronic PLP in people with amputations have been criticized for their associations with similar side effects [38, 48]. In contrast, a highly concentrated topical capsaicin patch applied to the amputation stump was found not only to reduce the intensity of both chronic pain at the site of the stump and PLP from the missing limb, but also to help a patient’s brain recognize that the limb where they once felt pain is gone [38, 48]. For people who have had leg amputations, attempting to use prostheses can lead to more stump pain when walking [38, 48]. Capsaicin is therefore beneficial for reducing pain while also enabling amputees to use prosthetics during the rehabilitation process [38, 48]. Ultimately, topical capsaicin offers a novel alternative with no significant adverse effects relative to traditional pharmacological treatments for pain management in people with amputations [38].
In a single bite of a spicy meal, the blazing heat from capsaicin molecules can not only ignite the sensation of fiery pain but also spark our understanding of unique pain treatments. By activating TRPV1 receptors, capsaicin triggers a cascade of pain signals that engage the body’s autonomic nervous system, producing responses like sweating and increased heart rate [11]. Yet, paradoxically, the initial pain is often met with pleasure due to the release of endorphins and dopamine, explaining why many enjoy consuming spicy food [24]. Along with helping to build tolerance to spicy food, capsaicin’s ability to induce desensitization through pain pathway interference
underscores its therapeutic potential for neuropathic pain conditions such as diabetic neuropathy and phantom limb pain [3, 4]. By targeting pain directly in the affected areas, capsaicin-based therapies provide a novel alternative to conventional medications with fewer side effects and greater patient satisfaction [46]. So the next time you decide to turn up the heat with a fiery curry or hot salsa, embrace the burn while savoring the science behind it!
References on page 84
by Daniel Bader | art by Alexandra Adsit
In the early 1900s, ‘punch drunk syndrome’ was used to describe a pattern of unusual behaviors observed amongst boxers, including emotional volatility and cognitive impairments [1]. As awareness of the condition increased, the disease was more accurately renamed chronic traumatic encephalopathy (CTE) [1].
CTE is a progressive neurodegenerative condition resulting from various types of head trauma or injuries [2]. Neurodegenerative diseases are characterized by cognitive and motor deficits due to nervous system damage and neuronal death [2, 3, 4]. While CTE was originally documented in boxers, it has recently gained notoriety due to its prevalence in former National Football League (NFL) players, particularly following its identification in NFL Hall of Famer ‘Iron’ Mike Webster [5, 6]. After his 19-year career, Webster experienced a rapid deterioration in health that was characterized by persistent difficulty with attention, severe depression, and unexplained fits of rage, ultimately culminating in his death at 50 years old [5, 6].
CTE’s diverse range of symptoms makes the disease complicated to identify, and to this day, CTE remains diagnosable only post-mortem, or after death [3, 7]. Advancing our understanding of how CTE develops is paramount as the condition continues to affect athletes and non-athletes alike [3, 7].
Repeated exposure to head trauma, such as collisions in American football, can contribute to the onset of CTE [8]. In fact, the likelihood of developing CTE is thought to double with every additional 2.6 years of playing American football due to the prevalence of forceful contact to the head associated with the sport [8]. Frequent instances of milder impacts can also induce the development of CTE [9,10]. For example, a soccer player heading a ball might experience only a minor impact with each hit, but repeated occasions of these seemingly harmless blows could contribute to CTE development [9,10]. While popular media typically portrays repeated patterns of head
injuries as the only sources of CTE, the disease can also be caused by a single, severe impact, also known as a traumatic brain injury [3, 11, 12]. Importantly, a single traumatic brain injury can cause immediate, significant damage to brain tissue and surrounding blood vessels [13]. Long-term inflammation from the initial injury can continue to harm the brain tissue for years [11, 13].
Although CTE is commonly linked to athletes involved in contact sports, various risk factors can increase the likelihood of developing the condition [3]. For example, CTE is frequently observed in military veterans, as repeated blast damage can cause significant head trauma [3]. Maladaptive behaviors that often begin in childhood, such as headbanging and other forms of self-injury, can also increase the likelihood of developing CTE [14]. Following the onset of CTE, several factors influence the speed of progression and the subsequent age at which people begin to experience symptoms [15]. Alcohol and drug abuse can accelerate neurodegeneration in individuals with CTE, causing symptoms to manifest earlier in life [15]. Likewise, genetics can also play a role in the progression of CTE [15, 16]. The TMEM106B gene encodes for the TMEM106B protein, which contributes to the regulation of lysosomes in the brain [15, 17]. In cells, lysosomes digest cellular waste, recycle cell components, and manage neuroinflammation [15, 17, 18]. Neuroinflammation is a part of the brain’s natural immune response to injury, and prolonged inflammation is detrimental to brain function as it causes structural changes and neuronal cell death [18, 19]. Variants of the TMEM106B gene can affect the regulation of lysosome activity, potentially increasing neuroinflammation [15, 17, 18]. Since TMEM106B regulates brain inflammation and health, individuals with CTE who have unfavorable TMEM106B gene mutations may be prone to experiencing clinical symptoms earlier in life [15, 16].
CTE symptoms can be strikingly varied and often remain hidden for years, sometimes taking up to a decade to fully surface [20]. Many people with CTE experience chronic headaches, diminished cognitive function, and language difficulties [21, 22, 23]. CTE can also impact an individual’s behavior, often causing increased impulsivity and aggression, as observed in ‘Iron’ Mike Webster [7, 22]. Behavioral alterations are thought to arise from CTE-induced neuronal death in the amygdala, a brain region involved in processing emotional stimuli and regulating decision-making behaviors [24, 25, 26]. As CTE develops, mental health problems such as depression and suicidal ideation may also result from dysfunction in the brain[22, 27]. CTE is a progressive disease, meaning that older individuals typically experience more severe cases with different symptoms as the disease damages the brain further [3, 7]. Cognitive deficits such as memory loss and difficulty with attention are more prevalent in older individuals with CTE compared to younger ones [3, 7]. Parkinsonism, which describes the tremors and walking abnormalities characteristic of individuals with Parkinson's disease, is also common among older people with severe cases of CTE [1, 3]. CTE presents a complex array of symptoms that progressively worsen over time, affecting not only physical and cognitive functions but also behavior and mental health [1, 3].
CTE is characterized by physical and functional changes involving tau, a protein responsible for providing structural stability to the brain [3, 10, 28]. Tau plays a crucial role in the assembly and stabilization of microtubules, which are components of the cell essential for its development, maintenance, structure, and communication [28, 29]. CTE is classified as a tauopathy, an umbrella term used to describe neurodegenerative diseases characterized in part by the abnormal accumulation of tau in the brain [30]. A typical tau protein bound to a microtubule contains two or three phosphate groups, which are negatively charged molecules that influence protein folding and function [31, 32]. However, severe head trauma can damage axons — thin fibers involved in neuron communication — leading to the addition of excess phosphate groups to tau in a process called hyperphosphorylation [30, 33, 34]. Hyperphosphorylated tau detaches from microtubules and becomes
misfolded, altering the structure and function of the protein, preventing tau from being used in the cell [35, 36, 37]. As a result, hyperphosphorylated tau aggregates, or clumps together, to form abnormal clusters called neurofibrillary tangles (NFTs) [10, 30, 38]. The accumulation of NFTs destabilizes microtubules and disrupts communication between neurons, leading to neuronal death and ultimately contributing to cognitive impairment [10, 36, 37].
Neurodegeneration resulting from NFT aggregates is also a hallmark of other tauopathies, such as Alzheimer’s disease (AD) and Parkinson’s disease (PD) [1, 20]. Although the location and specifics of NFTs differ across CTE and AD, individuals with either CTE or AD experience cognitive impairments, including learning and memory deficits [1, 20, 39]. Furthermore, both CTE and AD are characterized by neuronal death and decreased production of proteins involved in neuronal communication [20]. The accumulation of hyperphosphorylated tau and subsequent NFT formation observed in CTE are also features of PD [10, 39]. Additionally, there is a positive correlation between the concentration of hyperphosphorylated tau and the severity of PD symptoms like motor dysfunction and cognitive decline [10, 39]. While there is some overlap, the differences between AD, PD, and CTE are substantial, both in their underlying causes and in the specific symptoms experienced by affected individuals [1, 20, 39]. Nonetheless, understanding the common features these tauopathies share can help us understand more about tau-related neurodegeneration and guide the development of common treatment strategies [39].
Currently, there are many barriers that make CTE diagnosis challenging [3, 7]. Modern imaging technologies, such as magnetic resonance imaging (MRI), are not sensitive enough to detect NFTs in living people, so clinical diagnosis pre-mortem remains impossible [3, 7]. There are, however, some ways that CTE may be visualized in the brain after death [3, 7]. The locations of NFTs and the magnitude of neuronal damage, for example, are trademark indicators that can help differentiate CTE from similar tauopathies [3]. The current method of CTE diagnosis involves performing an autopsy of brain tissue to reveal the presence of hyperphosphorylated tau in brain regions such as the amygdala and hippocampus ([3, 7]. Similar tauopathies, such as AD and PD, are often also confirmed through post-mortem brain analysis, but clinical diagnoses can be made before death based on symp-
toms, neuroimaging, and biomarkers — indicators of disease that can be detected through medical testing [40]. Biological fluids like saliva, blood, and urine are commonly used for biomarker detection, as the presence of specific proteins or substances in these fluids can often be indicative of disease [41]. Since CTE has no established biomarkers and its symptoms, such as cognitive decline, mood changes, and tremors, are not unique to the disease, it is impossible to definitively diagnose the condition in living people [7]. Extensive research has been dedicated toward establishing diagnostic criteria for CTE in living individuals by assessing factors like mood, behavior, and cognitive function [2, 7]. However, such diagnostic approaches currently lack the necessary specificity and accuracy for reliably identifying CTE [2, 7]. Continued research into advanced neuroimaging techniques and biomarkers is essential to establish effective diagnostic criteria for detecting CTE in living individuals [2, 7].
Since CTE can only be diagnosed post-mortem, people suffering from CTE are often misdiagnosed [2, 21]. The misdiagnosis of CTE is common because CTE symptoms share common characteristics with numerous medical conditions [1]. For example, CTE is often mistaken for depression, as symptoms like anger, aggression, and impulsive behaviors — such as gambling and substance abuse — are common in both conditions [1]. Since CTE often manifests with cognitive decline and tremors, a misdiagnosis of AD or PD is also typical [2]. Detailed consideration of
medical history is of the utmost importance for CTE diagnosis, as seemingly inconsequential head trauma can have debilitating effects later in life [21]. Understandably, it may not be someone’s first instinct to attribute memory loss and motor impairment to a singular brain injury from years past, yet this disconnect is just another reason why CTE can go unrecognized [2]. Even if diagnosing CTE in living people were possible, such a diagnosis alone would not significantly improve symptom management or treatment [2]. Understanding the unique elements that contribute to the development of CTE on a case-by-case basis is beneficial because it elucidates potential risk factors of CTE, which can help prevent future cases [4].
Given the limitations of diagnosing CTE, treatments are often not specific to the disease itself and primarily focus on symptom management rather than preventing further damage in the brain [2]. Depressive symptoms associated with CTE are typically treated with a combination of antidepressants and mood-stabilizing medications used to treat clinical depression [2]. Likewise, motor disorders linked to CTE are treated with medications conventionally used to manage Parkinson’s disease [2]. The
utilization of depression and Parkinson’s medications in treating CTE demonstrates how CTE treatment often does not address the root cause of the disease, but rather attempts to alleviate symptoms to improve quality of life [2]. Treatments for similar diseases, such as AD, may be effective in CTE as they target a hallmark of the disease: hyperphosphorylated tau aggregates [1, 2, 20]. For example, kinase inhibition could potentially treat both CTE and AD by preventing excess phosphates from binding to tau [42, 43, 44]. Kinase enzymes moderate the addition of phosphate groups to proteins, so impeding kinase activity could prevent the accumulation of hyperphosphorylated tau by regulating phosphorylation [43, 44, 45].
Lithium salts, a standard treatment for bipolar disorder, have also emerged as potential agents for kinase inhibition [43, 44, 46]. Although it has not yet been tested in humans, research in animal models of AD has shown that lithium can prevent the formation of new NFTs and potentially prevent subsequent neuronal death [43, 44]. Promising treatments like kinase inhibition may offer potential strategies to slow CTE progression in individuals suspected to be living with the disease [43, 44].
While kinase inhibition and treatments aimed at alleviating symptoms of CTE have shown some promise, prevention remains the most effective strategy to combat the disease [45, 47]. Ice hockey has mandated the use of mouthguards in some high schools, which are believed to reduce the likelihood of concussion by 57% [48, 49] Other contact sports such as American football, rugby, and soccer have implemented soft shell padding to reduce head impact and the incidence of concussions [47, 50]. The NFL in particular has faced significant criticism for its lack of proactive measures regarding player safety [51]. For years, the NFL attempted to downplay and deny the connection between its players and CTE, only finally acknowledging the connection in 2016 [51, 52]. The NFL has since been forced to make changes to the sport to improve player safety and reduce head trauma, though concerns about transparency and integrity of the NFL’s handling of CTE remain [47, 53]. In 2022, the NFL introduced the Guardian Cap, a soft padding placed over the exterior of helmets designed to decrease the amount of impact force absorbed by the helmet [47]. Players in high-risk positions for repeated head trauma were required to wear Guardian Caps during the 2024 preseason, and the NFL reported a 50% reduction in concussions during that period [47]. However, clinical research regarding the Guardian Cap tells a different story, as impact testing has revealed that the device does not significantly reduce the force of head impact [47, 54]. Although the data do not directly dispute concussion incidence, it is clear that the NFL publicizes that the Guardian Cap has significantly greater efficacy than what is supported by clinical research, again calling into question the league’s supposed devotion to player safety [47].
Apart from attempts at physical protection, the NFL has also implemented concussion protocols to promote player safety [53]. For example, the NFL recently installed guidelines to prioritize accurate diagnosis of concussions and prevent injured players from
returning to athletic activities until they are medically cleared [53]. Additionally, ‘spotters’ are employed to monitor games and utilize replay review to identify players displaying concussion-like symptoms, which could indicate a head injury [53]. Rule changes have also been introduced to discourage head-to-head contact, such as the ‘targeting’ rule, which penalizes defensive players for initiating contact with their helmet when making a tackle [55]. As a result of these rule changes, equipment variations, and other preventative measures, the NFL reported a 17% reduction in concussions in the 2024 season [56]. However, this statistic notably does not exist outside NFL publications and was not subject to common scientific publishing standards, raising questions about the validity and integrity of the data [47].
CTE is a complex and devastating disease with widespread implications for those at risk, which includes anyone who has experienced a significant head injury or patterns of mild brain trauma [3, 11, 12] Individuals with CTE often develop severe cognitive impairment, motor issues, and headaches, as well as personality changes such as increased aggressiveness, depression, and even suicidal ideation [7, 21, 22]. Unfortunately, CTE often goes undetected due to its overlapping symptoms with other conditions and the lack of effective diagnostic criteria for living individuals [7]. Limitations in diagnosis and the lack of treatment strategies render CTE irreversible once established in the brain, so advocating for CTE prevention in the sports world and beyond remains paramount [2, 7]. Increased CTE awareness pushed the NFL to enact significant changes to rules and equipment, although the effectiveness of the alterations remains inconclusive as questions continue to surround the integrity of the NFL and its data claims [51, 54]. Changes to safety regulations in notoriously concussion-heavy sports are necessary and have considerable merit, but concussions in everyday life through car crashes, military service, and ordinary accidents will persist in the future [3, 57]. Therefore, efforts to address CTE in the future must include a multifaceted approach, including advocating for preventive measures, continued research into diagnostic systems, and further studies regarding CTE treatment plans [2, 22, 47].
References on page 86
DR. KATHLEEN SUSMAN, PHD
by Shawn Babitsky
Professor Susman’s journey into neuroscience wasn’t a straight line, but rather a winding path shaped by curiosity and circumstance. She began her journey at the College of William & Mary, with a strong interest in music as well as a fascination with animal physiology and endocrinology. She started out in chemistry, took a brief interest in psychology, and finally landed in biology. Her senior research project involved chemically separating compounds from mouse urine in an attempt to isolate pheromones that influence reproductive development. Susman thought, “maybe I’d discover the pheromone that triggers male development. Spoiler: I didn’t. But I did discover that tap water was stimulatory to reproductive development, which tells you something about how science always surprises you.” In graduate school at the University of Wisconsin–Madison, she rotated through a neuroscience lab and discovered her passion for neurobiology. But as much as she loved the research, there was something else calling to her: teaching. After her postdoc, Susman joined Vassar’s faculty and found that it was “the exact kind of environment I needed — collaborative, experimental, [and] student-focused.” While continuing her research in stroke neurochemistry, Professor Susman began to look at science through a more interdisciplinary lens and sought to bring science communication to Vassar. In her time here, she helped shape the neuroscience curriculum itself. She developed courses — like the (now) introductory neuroscience class required for all majors — that have become cornerstones of Vassar’s neuroscience program, and helped transform the Neuroscience & Behavior program from a small, siloed effort into a sprawling interdisciplinary initiative.
Around the same time, her research took a turn toward sensory neuroscience. She began working with a species of worm known as C. elegans to investigate how pesticide exposure affects mechanosensory neurons. Her lab began to show that common environmental chemicals can interfere with neuronal signaling — a finding with implications far beyond nematodes [1]. A pivotal collaboration with Professor Janet Gray deepened her interest in environmental science. Together, they launched a student-faculty journal club focused on endocrine disruptors and their effects on health “because we just wanted to learn more,” Susman says. “But it became this really powerful interdisciplinary space. We had students
from all different majors — neuroscience, environmental studies, political science — all bringing different questions to the table.” That marked the beginning of Susman’s transformation from research scientist to science communicator and activist.
She began working with local and state legislators, advocating for stricter regulations on environmental toxins and better public access to scientific information. “I realized I could leverage my scientific knowledge in places where it actually matters — where it can make a difference,” she says. Professor Susman utilized her knowledge of pesticides and partnered with the Natural Resources Defense Council (NRDC) in support of the Birds and Bees Protection Act, which was a groundbreaking New York State bill that bans the use of neonicotinoid pesticides that have been shown to harm pollinators and disrupt ecosystems. At the local level, Susman serves on the Environmental Management Council for the Dutchess County Legislature, where she’s taken on a lead role in addressing local environmental health threats. She’s especially passionate about raising awareness around PFAS, a group of man-made “forever chemicals” that have been found all over the world, including along the Hudson. And while navigating the legal and bureaucratic language of county reports, she brings the same urgency and accessibility to her writing as she does to teaching. “My goal is always to connect the dots,” she says. “To make the science readable and real. Because if you can’t explain to your neighbor why PFAS in the water is a problem, how can you expect them to care? Or vote?”
One of the biggest barriers to effective science communication, Susman believes, is the language of science itself: “The jargon we use in science can be alienating. It’s not that scientists are intentionally being elitist; it’s that the language can create a real disconnect between us and the public.” She points out that many students come to college with an aversion to science because they’ve been taught to believe it’s inaccessible or too difficult. “I’ve had students proudly tell me they’ve never even been in a science building during their time here. That’s a huge problem,” Susman says, shaking her head. “Science education shouldn’t just be about technical knowledge. It should be about making science something people can engage with, no matter their background,” she explains. In fact, she’s been pushing for years to teach a course that would blend science with literature. “I’ve been trying to get approval to teach a class called Biology in Translation for 15 years,” she laughs. “It would explore how figures like Thoreau used their understanding of nature and science in ways that made them meaningful to the public. I want students to understand that science isn’t just something we do in labs; it’s something that’s deeply connected to how we live our lives.” Ultimately, Professor Susman’s vision is clear: she wants to see a future where science is no longer something reserved for experts but something that belongs to everyone. “It’s not just for the privileged few; it’s for everyone.” As our conversation winds down, it’s evident that Susman’s work, both in the classroom and beyond, is driven by a deep commitment to making science something that everyone can engage with and understand. “Change doesn’t happen overnight,” she reflects. “But we’re starting to see some real momentum. Every small step forward is part of a much bigger movement.” And for Professor Susman, that’s enough to keep pushing forward.
Refrences on page 89
by Kyle Benson
Growing up in a geodesic dome in the rural farming community of Klamath Falls, Oregon, Professor Hadley Bergstrom’s early experiences with rugged surroundings sparked his fascination with how humans and animals interact with their environment. Long before he began decoding neural circuits of fear and memory, Professor Bergstrom spent his college summers fighting forest fires, pouring concrete foundations, and bagging ice for county fairs and convenience stores. Despite being drawn to cognitive processes and their neurobiological mechanisms during his undergraduate years at the University of Oregon, Professor Bergstrom was unaware of prestigious summer research positions — opportunities that today’s students often seek as stepping stones into academia. After spending a year abroad in Prague following his bachelor’s degree in psychology, Professor Bergstrom returned to the U.S. and settled in Portland, Oregon, where he began working as a research technician in a behavioral genetics lab at Oregon Health Science University studying addiction. Professor Bergstrom pursued his MA and PhD at George Mason University, where he investigated the impact of alcohol and nicotine on the brain. During this time, Professor Bergstrom began to understand addiction as a disorder of basic learning and memory processes, and would later dedicate his neuroscience career to understanding the neural mechanisms of learning and memory, with an emphasis on the profound impact of emotional processes on every aspect of memory, including formation, storage, and remembering.
After earning his PhD, Professor Bergstrom’s fascination with how emotion influences learning and memory led him to the Walter Reed National Military Medical Center in Washington, DC. During his post-doctoral fellowship at Walter Reed, he worked on mapping the topography of functionally active neurons associated with learning [1, 2, 3]. These pioneering preclinical experiments addressed novel questions surrounding the impact of trauma on learning and memory, such as Post-Traumatic Stress Disorder (PTSD). During this training stage, Dr Bergstrom also explored the use of paramagnetic contrast agents to improve high-resolution magnetic resonance imaging (MRI) scans [4]. He then transitioned to his second postdoctoral position at the National Institutes of Health (NIH) in the lab of Dr. Andrew Holmes, where he expanded his methodological toolkit by working with cutting-edge
technologies such as optogenetics, voltammetry, and advanced imaging techniques. At the NIH, Bergstrom investigated the roles of the basal ganglia and prefrontal cortex in visual discrimination tasks in mice using touchscreen-based systems [5]. This research employed advanced techniques to manipulate and monitor brain activity during cognitive tasks, further shaping his interest in how neural systems regulate learning and decision-making — particularly concerning stress and addiction. Since joining Vassar College in 2015, Professor Bergstrom’s research has focused on how the brain utilizes past experiences to guide behavior in uncertain situations, especially through a psychological process known as stimulus generalization. Using chemogenetics and activity-dependent tagging, his team recently identified a population of neurons — an engram — in the infralimbic prefrontal cortex whose activity suppresses generalized fear responses [6]. Their findings provide new insights into how the brain distinguishes between real, ambiguous, and no threat, with significant implications for understanding anxiety and PTSD.
Professor Bergstrom has developed a passion for making complex neuroscience topics more accessible to students. He believes that while neuroscience can often seem overwhelming due to its intricate nature, breaking down complicated ideas into understandable concepts is essential for fostering curiosity and comprehension. In his research methods in physiological psychology course, Professor Bergstrom exposes students to issues related to the ethics, design, measurement, analysis, and reporting of research and laboratory topics. Implementing his approach to presenting results in his many research studies, Professor Bergstrom emphasizes the importance of making results and figures understandable to any reader, whether or not they have a background in the topic. As Professor Bergstrom is dedicated to fostering interest and engagement in neuroscience among the next generation of scientists and researchers, he seeks to organize a neuroscience conference at Vassar, bringing together Neuroscience & Behavior professors from liberal arts colleges nationwide to enhance the overall Neuroscience & Behavior program.
References on page 89
by Kate Billow
Neuroscientific research is happening all over campus, even where you wouldn’t expect it. Tucked away in the anthropology department, in the basement of Blodgett, is where Professor Zachary Cofran studies the fossil record in the exploration of human evolution. Specifically, he looks at endocasts — fossilized fragments of the internal surface of the skull which can contain impressions left by the brain — to hypothesize about the evolution of the brain as well as growth and development in ancient hominin species. His office is covered in images of brains, fossils, and 3-D prints of the bony labyrinth, the cavity containing your inner ear organs. At one point during our interview, he hands me a tiny 3-D printed scapula, the bone that connects the upper arm bone to the collarbone, from an extinct hominin called Homo naledi. I turn the fragment between my fingers, unsure how this tiny, nondescript bone could reveal much about human evolution. As it turns out, it is these tiny fragments and a handful of well-preserved fossils that tell us nearly everything we know about human evolution. Unfortunately, soft tissue like the brain doesn’t fossilize. So when reconstructing our evolutionary divergence from our closest living relatives, the great apes, we must rely on these seemingly insignificant bits of bone to learn about the origins of the profound functional differences (and surprising similarities) between modern humans and great apes. Professor Cofran explains, “The big challenge in our field, really, is we have this fragmentary evidence for what life was like, what animals were like, what humans were like in the past. And it will tell us something about how we got here as a species, but it is very hard to actually extract that information. So what we really do in the field is use the fossils as a basis for addressing these questions in living animals.”
Originally, Professor Cofran had thought he would pursue Greek and Roman studies when he took an anthropology class during undergrad at Loyola University Chicago. His first anthropology class sparked his interest in the intersection between culture, language, archaeology, and biology, specifically: “how we got here.” He explains, “we are unique in how just utterly bananas we go about doing things. And that’s where the evolutionary stuff comes in. Like, how on Earth did we get here?” Over the course of his career, including nine years at Vassar, he has sought and studied fossils from across the globe — Kazakhstan,
South Africa, and Croatia — and studied specimens from many species, including Homo naledi, Australopithecus, Neanderthals, Homo erectus, and modern gibbons. Gibbons are a diverse type of ape living in southeast Asia and can serve as a valuable comparative model when looking at the evolution and diversity of hominin brains in the fossil record: “The big question is, does this [anatomical diversity] relate to the underlying brain itself and what it's doing and how it's impacting how these animals interact with their environments? Is there a brain difference that we can see in the fossil record that can tell us something about the emergence of culture or intelligence?”
We talk about how neuroscience, anthropology, and archaeology are all intertwined in a desire to understand ourselves, our species. Again, fundamentally, “how we got here.” How did we get from great apes to Neuralink? He elaborates, “it’s a cool puzzle, and the fossils are just kind of a way to start asking these questions, I think.” I’m in Professor Cofran’s anthropology seminar this semester, Is the Human Brain Special? We delve into this question, and discuss how interdisciplinary work in anthropology and neuroscience, such as his, can help to address this fundamental question: “Human diversity is so low in terms of like the actual inherited genetics, even compared to other animals. But when you look at the fossil record, wow, you've got a bunch of very different kinds of humans running around, probably interacting with one another for literally millions of years up until probably about 40,000 years ago. And so at the very least we can say, look, we're not that different.” He points to his computer screen, swinging it around so I can see his wallpaper, a fragment of a Homo naledi skull whose brain and inner ear he’s studying. “That was very different.”
by Evelynn Bagade
Dr. Lori Newman is on the lookout for novel connections and alternative explanations. Throughout her career as a neuroscientist, Dr. Newman has sought to understand the function of astrocytes — the unique star-shaped cells of the nervous system — and their influence on brain health and disease. By remaining open to new hypotheses about the role of these cells, she has not only succeeded in furthering our understanding of astrocytes’ key role in metabolism, but has also inspired her students to stay curious and keep asking questions. Dr. Newman’s own interest in science began at a young age. As a high school student, she originally wanted to pursue a career as a marine biologist and began working on a project focused on dinoflagellates, a type of plankton that form symbiotic relationships with coral. After high school, Dr. Newman left her hometown in upstate New York to attend The College of William and Mary in Williamsburg, Virginia. Dr. Newman unlocked “a whole new world” of complexities when she took a physiological psychology class and committed to studying neuroscience. In pursuit of gaining hands-on experience, she began working as a research assistant in a lab at William and Mary that focused on investigating the role of the intralaminar nuclei of the thalamus in sleep-wake cycles and attention in rats. Dr. Newman then matriculated into a combined MA/PhD program at the University of New Hampshire, where she studied neuromodulatory systems and proposed a behavioral test that could be used to study cognition in rats for her thesis.
It was not until she began a postdoctoral position at the University of Illinois Urbana-Champaign, that Dr. Newman began to narrow her focus on astrocyte cells. At this point in time, it was generally understood that astrocytes were a type of glial cell — cells that play a role in supporting neurons — but the specifics of how the astrocytes carry out their supportive functions were not well researched [1]. In Dr. Newman’s opinion, if the astrocytes were present in the nervous system, they must serve a specific purpose, and she ultimately decided to study how astrocytes could potentially work as “shuttles” that provide other brain cells with the materials they need to function. Dr. Newman explains that astrocytes are exciting to study because they aid in maintaining the brain’s connection to the rest of the body and make neurons ready to do their work. While neurons send
and receive chemical messages, astrocytes “listen” to the neurons and deliver essential nutrients, such as glucose and oxygen, from the blood to the neurons when necessary [2]. Without the support of astrocytes, neurons would not be able to receive the energy and nutrients required to function [3]. Given that one of their primary roles is to help neurons, astrocytes are thought to be crucial in the first line of defense against injuries [4]. Furthermore, glia are thought to play a role in neurodegenerative diseases if they become dysfunctional, making these cells important to research [5]. Out of the many purposes that astrocytes serve, Dr. Newman is ultimately interested in determining how astrocytes support metabolism, the process by which cells use energy to carry out essential functions, within the brain.
In her current role as a professor and researcher at Vassar College, Dr. Newman investigates astrocytes in rats while training undergraduate students in her lab. The Newman Lab is currently working on multiple projects; for example, one project is investigating the effects of the ketogenic diet on astrocyte function, and another is focused on how astrocytes could play a role in sustained attention. Within the lab, Dr. Newman encourages her research students to not only learn the important benchwork and analysis skills, but also to think critically and have the “tenacity to question.” Dr. Newman views research as a continuous process, as scientific inquiry does not stop after just one question is answered. Rather, she believes it is important to keep asking questions and be open to considering alternative answers. Thinking back to her own time spent working as an undergraduate research assistant at William and Mary, Dr. Newman says that being able to ask questions, grow from her mistakes, and learn from graduate student mentors was a formative experience; in fact, she continues to collaborate with some of her college labmates to this day. By encouraging underclassman lab members to shadow upperclassmen labmates, her students can get involved in the lab community and prepare to mentor other labmates themselves in the future. Going forward, Dr. Newman holds the same hopes for both her students and the neuroscientific field as a whole: that research will continue to become interdisciplinary and collaborative, and become more inclusive of novel perspectives.
References on page 90
by Julia Fallon
Dr. John Long has been a Vassar faculty member since 1991 as a professor in the Cognitive Science, Biology, and Neuroscience and Behavior departments. Ever since he was young, Dr. Long was interested in being underwater: he swam a mile to graduate high school in Michigan, consistently watched Jacques-Yves Cousteau and his self-contained underwater breathing apparatus (SCUBA) on television, and ended up at the United States Coast Guard Academy. Dr. Long often says, “I love fish and I am a fish,” as he has found his passions in the intersection between the ocean and biology. Dr. Long attended the College of the Atlantic on a little island off the coast of Maine, and proceeded to earn his PhD in biomechanics from Duke University. During his graduate studies, Dr. Long recognized his interest in connecting two key parts of science: behavior and biological systems. Dr. Long’s research interests emerged from bridging the gap between musculoskeletal biomechanics and behavior through the lens of neuroscience.
At Vassar, Dr. Long has spent much time researching skates — a flat, pancake-type of cartilaginous fish related to a manta ray. Cartilaginous fishes, like rays and sharks, have evolved to be boneless [1]. Currently, a team of undergraduate students observes the skate embryo and studies its behavior and energetics as it spends close to a year inside its egg capsule with only three grams of nourishment. Dr. Long’s research attempts to answer how three grams of nourishment can be enough to live and grow for 9-12 months. Another group of researchers in Dr. Long’s lab is exploring how anthropogenic noise, such as boat noise, may stress embryos by causing them to freeze and potentially disrupt their vital functions. Dr. Long’s research ties into broader questions about the evolution of bone loss in cartilaginous fishes, which offers insights into vertebrate evolution and the ecological factors that shape these creatures. Dr. Long emphasizes the importance of curiosity-driven research in fields that may not have immediate or obvious benefits for humans. In his experience, it's often the projects with no clear benefits that have the most rewarding outcomes.
When translating his research into a simpler explanation for a more general audience, Dr. Long likes to find a bridge through which he can connect difficult scientific subjects to general knowledge. For
example, when Dr. Long presents his research on skates, he compares them to manta rays to help people understand the type of fish that he is studying. One of the biggest barriers to understanding neuroscience, according to Dr. Long, is when you get into any sort of information that requires foundational work. He finds that when a student expresses dislike of a subject or says they aren’t good at it, it's often due to a negative experience with the subject — like not meshing with a teacher — rather than a lack of ability. For students who feel this way, Dr. Long encourages them to give themselves room to explore interests and paths without being afraid of making a mistake. Science is supposed to be collaborative and exciting, and also requires trial and error to find something you are passionate about.
References on page 90
DR. BOJANA ZUPAN, PHD
by Eve Andersen
Professor Bojana Zupan’s journey into the world of neuroscience began with a simple but profound curiosity: how does the brain shape behavior? She decided to only apply to colleges which offered a major in biopsychology, which was what the study of neuroscience was frequently called at the time. At Barnard College, she became intent on “learning why animals do the things they do,” which informed her initial focus on animal learning and behavior. However, a research methods class in physiological psychology in her junior year exposed her to the world of brain circuit manipulation. She learned to perform stereotaxic surgery on rats to manipulate specific brain regions to elucidate their impact on animals’ behavior. Professor Zupan became fascinated with a molecular and systems approach to neuroscience rather than just behavior itself. She was amazed at how much more could be discovered when you are able to “tinker under the hood” of the brain. This led her to pursue a PhD at the Weill Cornell Graduate School of Medical Sciences, where she researched the genetic and environmental impacts of maternal mutations on offspring behavior.
After completing her PhD and spending an additional year at Weill Cornell as a postdoctoral research fellow. Then, Dr. Zupan left academia to pursue a related passion, explaining science to a broad audience. Professor Zupan went to work as the Assistant Medical Editor at ABC News where she helped research and write content for health and science-related stories for the Network and its affiliate stations across the US. However, after a year, Professor Zupan’s undergraduate advisor encouraged her to revisit academia at a liberal arts college where she would get an opportunity to teach undergraduates while still performing the research she loved. Professor Zupan left ABC News to take a year-long position as a Visiting Assistant Professor at Bowdoin College. Although initially unsure if she would enjoy teaching undergraduates, Professor Zupan found the experience deeply rewarding. She loved seeing her students become excited about the same ideas she had also been fascinated with since she was a teenager. Teaching also allowed Professor Zupan to guide students through a research methods class similar to the one in her undergraduate years that exposed her to the world of circuit manipulation and informed her research career.
After her year at Bowdoin, Professor Zupan landed a tenure-track position at Vassar College where she has since remained. At Vassar, Professor Zupan runs an active research lab that investigates the relationship between reward circuitry and social behavior in mice. Mice, like humans, continue to engage in social behaviors if the experience is rewarding [1]. Reward informs motivation. For example, if an experience is rewarding to an animal, that animal will be motivated to pursue that experience in the future. Reward, and therefore motivation, is thought to be encoded by dopamine neurons in the brain [1]. Currently, Professor Zupan is examining how the activity of dopamine neurons in the ventral tegmental area — an origination point for many dopamine neurons — is affected when a mouse has social interactions. Her lab is able to record the activity of these neurons in the brain while observing the natural behavior of mice.
Dopamine, reward, and motivation are thought to be dysregulated in certain neurodevelopmental disorders like Autism Spectrum Disorders (ASD) [1]. People with ASD show deficits in social communication and social interaction, which may be due to the dysregulation of social reward networks [1]. Therefore, Professor Zupan’s lab is specifically looking at the activity of dopamine neurons in a mouse model that lacks the FRM1 gene, which in humans is a common site for mutations associated with ASD [2]. Similarly to people with ASD, the mouse model also displays some social deficits in behavior [2]. Professor Zupan’s research seeks to establish whether the activity of dopamine neurons in the VTA contributes to observed differences in sociability. Her research has the potential to inform the understanding of the mechanisms behind social deficits seen in disorders like ASD and more broadly improve understanding of social behavior.
References on page 90
by Chloe Bilger | art by Elizabeth Catizone
Wrought with grief and arms heavy with his daughter’s slain body, the mad king was so devastated that he died of a broken heart — or so the story goes. In Shakespeare’s tragedy, King Lear, King Lear banishes his daughter Cordelia after she fails to proclaim her familial love for him. However, after a deceitful betrayal by his two other daughters, the King himself is banished and searches for Cordelia in an attempt to reconcile with her. Tragically, he finds his beloved daughter dead. The King’s heart breaks at the sight of her body, and in an instant, he dies.
Since the 1600s, dying from a broken heart has been seen throughout the classics as a mythical tragedy, striking down great protagonists for centuries [1]. Yet, despite its cultural prevalence, a ‘broken heart’ has only been recognized as a medical condition for the past 30 years [1]. Today, we can interpret King Lear’s tragic death as a case of Takotsubo Syndrome (TTS), also known as Broken Heart Syndrome, a condition where the heart is thought to be physically damaged by one’s emotional stress [2, 3].
First introduced in Japanese literature in the 1990s, TTS is a unique heart condition often initiated by an intensely stressful event, such as the death of a loved one [3, 4]. Until recently, the origins of TTS were masked by its striking resemblance to a heart attack, as both conditions cause chest pain, shortness of breath, and changes to the heart’s rhythm [5, 6, 7]. As a result of overlapping symptoms, an estimated 7,000 to 14,000 Americans with TTS may be misdiagnosed each year, preventing those individuals from receiving proper care [8, 9]. However, despite significant similarities, the underlying mechanisms that incite a heart attack and TTS are particularly different [10, 11]. Heart attacks are often induced by a build-up of fat, cholesterol, and calcium called plaque, which forms along the walls of the coronary arteries that supply blood and, thus, oxygen to the heart [10, 11]. Over time, the plaque can obstruct blood flow to the heart, making it strenuous for the heart to pump blood and eventually leading to a heart attack [10, 11]. In contrast, during TTS, the heart’s left ventricle — the largest chamber of the heart — spontaneously ‘balloons’ or expands outward, causing the ventricle to be temporarily disfigured [11, 12]. The ballooning of the left ventricle in TTS prevents the heart from adequately pumping blood, leaving the heart temporarily dysfunctional [11, 12]. The shape formed by the expanded left ventricle bears a striking resemblance to a traditional ‘Takotsubo’ pot used to catch octopuses in Japan, giving TTS its name [13]. The leading
hypothesis behind the ballooning of the left ventricle in TTS suggests that dysfunction in the nervous system’s response to stress is the underlying cause [11, 12]. As a result, understanding how emotionally traumatic stressors induce TTS may illuminate currently unexplored neural connections between the brain and the heart.
The nervous system coordinates a physical response to cope with environmental stressors, like the death of a loved one [14]. The brain activates the sympathetic nervous system (SNS), which is a branch of the nervous system responsible for triggering the fightor-flight response [15]. The SNS can be best understood as the ‘emergency system’ and, once activated, sounds the alarm by releasing catecholamines, a group of hormones and neurotransmitters that increase the body’s heart rate and blood pressure to prepare the body for potential dangers [7, 16]. Catecholamines involved in TTS include epinephrine, more commonly known as adrenaline, and its precursor molecule, norepinephrine [7, 16, 17, 18, 19]. Normally, stressful events trigger a stress response characterized by the activation of the SNS and the subsequent production of catecholamines [7, 17]. However, in TTS, there is an overactivation of the SNS, leading the brain to send signals to overproduce epinephrine and norepinephrine [17]. The excess of catecholamines creates a type of chemical toxicity that directly damages the myocardium, the muscular layer of the heart that contains specialized heart cells responsible for generating the heart’s contractions [17, 20].
Catecholamines can also indirectly cause damage by promoting constriction of the coronary arteries [17, 20]. When epinephrine and norepinephrine flood the nervous system, they bind to receptors on the coronary arteries, causing the arteries to constrict and thus reducing blood flow and oxygen to the heart [17, 20]. Additionally, the overproduction of catecholamines in TTS makes the heart contract even more frequently, resulting in an imbalance between how hard the heart is working and how much blood is actually being supplied to the heart [17, 20]. Essentially, the tissues in the heart are not receiving enough oxygen for their workload, which can also lead to damage to the myocardium [17, 20]. The myocardial damage may lead to ‘myocardial stunning’ — a dysfunctional state of the heart characterized by a decrease in the heart’s ability to contract and subsequently pump blood [17, 20]. Myocardial stunning may result in the outward ballooning of the left ventricle, forming the Takotsubo pot-like shape, thus causing heart dysfunction [12, 17, 21]. Therefore, if emotional stress facilitated by catecholamines is powerful enough to induce heart dysfunction, investigating TTS may elucidate the connection between emotional processing and physical health [1, 22, 23].
When the brain responds to stressful triggers, the SNS is activated by a network of brain regions involved in emotional processing and mediating cardiac responses to emotional stimuli, referred to as the central autonomic network (CAN) [14, 24, 25]. The CAN activates the SNS to carry out stress
responses, highlighting a potentially important relationship between the CAN and TTS [14, 24] One important component of the CAN is a brain structure called the insular cortex (IC), which integrates emotional stimuli and stimulates the nervous system to generate a physiological response [14]. The IC is primarily responsible for emotional processing and, as such, helps assign emotional valence or the emotional connotation to an experience or event [14]. Interestingly, studies involving neural lesions — controlled damage to brain tissue — have further illuminated the connection between the IC, excess norepinephrine production, and thus TTS [26, 27]. To determine which neural structures are involved in heart dysfunction, neural lesions were induced in various brain regions, including the IC [26]. Lesions in the IC result in the release of norepinephrine and subsequent heart dysfunction, with more severe lesions correlating with higher release of norepinephrine and more severe dysfunction [16, 24, 26, 27, 28]. The correlation between lesions in the IC, norepinephrine release, and heart dysfunction suggests that dysfunction in brain regions of the CAN may play an important role in TTS [26, 27].
The CAN also includes the amygdala, a structure that is similarly crucial in processing stressful emotions [29]. The amygdala helps assign negative emotional valences to experiences and manages the body’s stress response through the nervous system [25, 29, 30]. Ideally, stress responses are facilitated by communication between the CAN and the SNS, leading to catecholamine production and other physiological responses [24]. However, in TTS, communication between regions in the CAN may be disrupted by alterations in functional connectivity — levels of simultaneous neuronal activation between disparate brain regions — within the CAN [9, 25, 31]. Interestingly, TTS has been linked with both increases and decreases in insular-amygdala functional activity, indicating that deviation in either direction may result in dysfunction [9, 25, 29]. In TTS, alterations in connectivity between brain regions like the IC and amygdala may then instigate the overactivation of the SNS and thus overproduction of catecholamines [9, 17, 24, 25]. Suppose we consider the cause of King Lear’s death to be TTS: the overwhelming stress from the death of his daughter served as the stressful stimulus [14]. King Lear’s CAN works to process the emotional burden and initiates the stress response throughout his body [14]. Consequently, King Lear’s SNS was overactivated, leading to an excessive release of catecholamines, possibly causing the ballooning and left ventricular dysfunction that is characteristic of TTS [17, 32].
The tragic death of the titular character in Shakespeare’s King Lear presents a timeless and compelling narrative that may, in fact, have its roots in a real and medically recognized phenomenon: Takotsubo Syndrome (TTS). While King Lear himself seems to be a relic of old literature, his heartbreak remains a real and prevalent issue. Modern science has now uncovered that the emotional distress experienced in moments of extreme grief or stress can have physiological effects, leading to the cardiac dysfunction seen in TTS [17]. The critical intersection of emotion, the brain, and the heart urges a deeper investigation into how emotional stress can manifest itself in profound physiological consequences [2, 3]. The study of TTS offers valuable insight into how the brain processes emotional pain and how this can trigger a cascade of events that ultimately disrupt the heart’s physical functioning [11, 17].
Furthermore, altered brain connectivity observed in TTS cases suggests a potential disruption in the brain's emotional regulation systems in response to extreme stress, offering an exciting avenue for future research [9, 25, 29]. Uncovering the mechanisms governing TTS not only deepens our understanding of the syndrome but also encourages a broader exploration of how emotions influence physical health [1, 22, 23]. As scientific knowledge of TTS evolves, it could pave the way for more effective treatments for individuals suffering from both emotional and physical trauma, emphasizing the need for integrated approaches to emotional and cardiovascular health [11]. In Act III, King Lear laments, “Is there any cause in nature that makes these hard hearts?” As it turns out, there is [33].
References on page 90
by Layla Fakki | art by Emily Holtz
After a long day at work, you return home to find water pooling at the foot of your bathroom sink. Your heart sinks as you open the cabinet under the sink and find that a pipe has been slowly leaking. You quickly scramble to clean up the mess, but something feels odd as you start mopping the floor. There is a faint, musty smell in the air, something you can’t quite place. You think it’s just the dampness at first, but as time passes, the lingering smell grows stronger and more unsettling — until eventually it disappears. Then you start to see the dark green spots on the walls. You go from room to room, inspecting every nook and cranny, discovering more and more mold. What started as a slight inconvenience has become something much worse. Stachybotrys chartarum, known colloquially as black mold, is far
more than just the dark-colored fungus that grows in the corners of your home [1]. Black mold exposure has been linked to various health problems, including fatigue, headache, persistent coughing, congestion, chest tightness, and loss of smell [2, 3, 4]. While it may be easy to dismiss a few black spots on your shower curtain, the insidious nature of black mold has the power to wreak havoc on your everyday life.
Black mold is one of the most common infectious fungi that grows indoors, distinguished from other household molds by its unique physical and chemical characteristics [4]. Though the fungus is named for its distinctive tar-like appearance, it can also appear green or dark brown, depending on its environmental conditions [3, 4]. The most crucial factors for black mold growth are high humidity and moisture, making areas affected by water infiltration, damage, or leaks ideal environments for its development [3]. Although black mold thrives in warmer temperatures, it can survive across a wide temperature range, from 36.5ºF to over 98ºF, allowing it to grow in diverse environments [3]. In order to survive, black mold feeds on cellulose, a sugar that provides structural support within plant cell walls [3, 5]. Drywall and wallpaper are examples of cellulose-rich materials found in homes that allow black mold to grow unseen [1, 6]. Think of the warm, moist environment ideal for mold growth like a middle school cafeteria: perfect for people to gather and for rumors to spread quickly. Similar to how pieces of information are overheard and passed between groups of students, black mold can diffuse through wallpaper and spread throughout an entire house, often going unnoticed until it is too late [6].
The small inconvenience that started in your bathroom took over your whole house through the specialized airborne reproductive cells of fungi called spores [7, 8]. Black mold spores can enter and harm the body via inhalation, ingestion, or direct contact [3,
9]. While one can avoid touching or ingesting moldy objects, it is much harder to protect oneself from inhaling airborne spores [3, 10]. With inhalation, black mold spores enter the body through the nose and release mycotoxins, which are small molecules produced by fungi that can pose a hazard to the health of other organisms [11, 12, 13]. The mycotoxins diffuse through the nasal epithelium, a protective tissue that lines the nasal cavity [9, 14, 15]. A large segment of the nasal epithelium, known as the olfactory epithelium, is located in the upper half of the nose and is responsible for detecting smells [16]. The olfactory epithelium contains olfactory sensory neurons that send signals to the olfactory bulb, the first part of the brain responsible for processing smell [17, 18]. The mycotoxins diffuse through the olfactory epithelium and use the olfactory sensory neurons to reach the olfactory bulb and subsequently the rest of the brain, wreaking all sorts of havoc [18, 19, 20].
Mycotoxins cause damage in essential bodily processes by disrupting the immune response and increasing inflammation [21, 22]. The most potent types of black mold mycotoxins are satratoxins, which increase the amount of free radicals in the brain [22, 23]. Free radicals are highly reactive molecules that can be neutralized by compounds called antioxi dants. [24, 25] When free radicals vastly out number antioxidants, the overwhelming imbal ance induces a destructive state that disrupts cellular function and communication referred to as oxidative stress [1, 3, 26]. The excess free radicals caused by satratoxins react with lipids, proteins, and DNA, and in the case of black mold mycotoxins, microglia [22, 24, 27, 28]. Microglia are one of the brain’s primary immune cells that play a key role in trigger ing neuroinflammation [29]. Inflammation is part of the body’s immune response against harmful stimuli, such as mycotoxins; when it occurs in the brain, it is known as neuroin flammation [28]. In oxidative stress, free rad icals activate microglia, which in turn activate proteins called mitogen-activated protein ki nases (MAPKs), increasing neuroinflammation and subsequently impacting neuronal func tion [27, 30, 31].
MAPKs cause two extremely damaging pro cesses: inflammation and cell death [21, 32]. Inflammation is helpful in the short term as it
helps the body respond to injury or infection; however, chronic inflammation can lead to tissue damage [28]. Inhaling the mycotoxin-containing spores from black mold over a long time leads to chronic inflammation and damages the surrounding nasal tissues [21]. Additionally, tissues are further damaged through cell death induced by the MAPKs [32]. The combination of cell death and neuroinflammation causes permanent damage to the body, especially in the olfactory system [21, 33]. A loss of smell stems from the death of olfactory sensory nerves as well as the atrophy of the olfactory epithelium and olfactory bulb [4, 33]. Prolonged neuroinflammation associated with black mold infection may increase susceptibility to neurodegenerative and sinus disorders [21, 33]. One proposed treatment avenue for black mold infection is supplementing an antioxidant known as glutathione [21]. Since glutathione deficiency is associated with the oxidative stress caused by black mold toxins, supplementing glutathione could neutralize the effects of satratoxins [21, 34]. Nasally administered glutathione has been found to reduce mycotoxin-induced neurocognitive symptoms [21]. Timely recognition and intervention are vital to mitigating the health risks associated with mold exposure and preventing further complications [35, 36].
Black mold has been attributed to a neurological disease known as sick building syndrome (SBS) [37]. A term first coined in the 1970s, SBS refers to a series of nonspecific respiratory and neurological symptoms experienced by a group of residents or coworkers who spend most of their time in a particular building [38]. Headache, fatigue, difficulty concentrating, dizziness, nausea, and irritation of the eyes, nose, and throat are common symptoms of SBS [37]. Individuals affected by SBS often feel a sense of relief from their symptoms soon after leaving the building [37]. Many possible causes of SBS exist, which may vary from building to building [39]. One potential risk factor is poor ventilation, as pollutants can accumulate indoors and be inhaled by unknowing inhabitants when buildings are inadequately ventilated [38,39]. A number of pollutants have been associated with SBS, including chemicals like formaldehyde and biological material like animal droppings [37]. Notably, the growth of black mold is also a prominent cause of SBS [37]. There is an association between the presence of indoor mold and SBS symptoms; visible mold has also been linked to increased SBS symptoms [40]. Targeting the source of the pollutants is the usual treatment for SBS, such as removing air ventilation filters, replacing water-damaged ceiling tiles and carpeting, and installing new filters to clean the air [37].
DON’T LET MOLD TAKE HOLD: AVENUES FOR FUTURE TREATMENTS
The discussion of black mold as a cause of neurological issues is relatively new, and further research is needed to understand how black mold affects the body [41]. The complex nature of mold exposure, individual variability in responses, and challenges with isolating mold-specific effects are some limiting factors regarding treatment [42]. Antioxidants, like glutathione, have been proposed as treatments because of their ability to counter oxidative stress and reduce neuroinflammation [21]. But these treatments are still in their infancy [21]. Some individuals who are experiencing symptoms from mold exposure have instead turned to unconventional treatments, including changing their diets, taking antifungal medications, and undergoing detoxification processes [36]. However, unconventional therapies tend to lack evidence of effectiveness [36]. Although many treatments have been proposed to combat satratoxins and their damaging effects, the most reliable remedy is prevention of exposure [36]. A common thread among proposed
solutions is the need to either eliminate the mold or remove oneself from the contaminated environment, whether by arranging a mold inspection of the living space or taking the opportunity to move [41]. Relocating, however, can present challenges in many situations, reinforcing the importance of early recognition to prevent the spread of black mold. Though research is scarce, every day we are learning more about black mold: how it spreads, how it damages the brain, and how it can be treated.
References on page 92
by Leo Mahlke | art by Racine Rieke
What is the first thing that comes to mind when you think of speech therapy? Maybe a child who says ‘thun’ instead of ‘sun.’ Perhaps you can imagine the child meeting with a speech-language pathologist (SLP) who guides them through repetitive drills and flashcards until their vocal fluency is improved. However, SLPs do much more than help with speech impediments; they work with people facing a diverse range of communication disorders and use a wide variety of treatment methods [1, 2, 3]. People with communication disorders can struggle to varying degrees with comprehending language, producing fluent speech, or communicating with others [1, 2]. While some are born with a communication disorder, others develop impaired language skills due to brain damage or age-related decline [4, 5]. Therefore, SLPs may work with anyone from premature babies with swallowing disorders to older adults with memory loss. To target the spectrum of needs for people with communication disorders, SLPs apply their broad expertise in neuroanatomy and physiology across diverse settings [4, 5]. Music is used as an innovative tool to support communication and language development [6, 7, 8]. Music activates brain regions associated with listening, moving, emotional processing, decision-making, prediction, and rhythm — all of which are essential for communication [9, 10, 11]. Engaging with music through activities like singing strengthens the relationship between auditory skills and motor responses, both of which are necessary for speech production [7, 12, 13]. Since the brain processes both music and language’s rhythms, melodies, and structures similarly, exposure to music can directly support language development [12, 14, 15]. The implementation of music into speech therapy can include playing lullabies for infants, rhythmic repetition of syllables, and group singing with elderly people [10, 12, 16, 17].
As hierarchical systems, music and language are built from smaller units that come together to form larger structures [18]. Like beads strung together to make a necklace, syllables combine to form words and sentences, and musical notes combine to create songs [18]. Baby babbling, an early stage of vocal development, is a prime example of how the smallest hierarchical units of language and music are shared [19, 20, 21]. Early vocalizations like ‘bah’ or ‘gah’ combine the smallest subunits in the language and music hierarchical systems [19]. The shared hierarchical structure may explain why infants initially rely on similar parts of their brains to process both language and music [19, 21]. As we move up the hierarchical system from individual units to sentences or songs, specialized
regions of the brain help us interpret their meanings [15, 18]. For example, Broca’s area, a neurological site of language processing and production, plays an important role in understanding the hierarchical systems of language and music [22, 23]. Broca’s area allows us to construct grammatically complex sentences and recognize nuanced musical patterns [22, 23].
Both music and language involve the brain perceiving, categorizing, and assigning meaning to sound [24, 25]. Sound identification and categorization depend on working memory, a form of short-term memory that allows people to retain and manipulate information even when absent from their immediate environment [26]. For example, if a child is taught and subsequently told to sing a new song, they use their working memory to remember the lyrics [26, 27]. Working memory also allows children to model their speech after those around them, so children with poor working memory are more likely to struggle with communication. If a parent prompts their child to repeat the word ‘Dada,’ a child with working memory deficits may struggle to remember and accurately repeat the sounds they just heard [26, 27].
Imagine how a sentence would sound if each word were one syllable and completely monotone. The sentence would feel flat and meaningless because no word would be particularly emphasized to convey the speaker’s intention [26]. Similarly, if every note in a song were the same, the song would feel repetitive and boring. When music and language lose their melody and rhythm, they also lose some of their contextual meaning [26]. Because language is inherently
rhythmic, pairing speech with the sounds of a steady rhythm can enhance one’s grammar usage through a phenomenon known as the rhythmic priming effect [28, 29]. For example, when a child listens to music with a predictable and regular tempo, they show immediate — though momentary — improvements in grammar and syntax due to the changes in their brain wave activity [28, 29]. Brain waves are patterns of electrical activity created by groups of nerve cells in the brain [30]. While listening to a steady beat, a child’s brain waves that are associated with prediction, speech, and motor outputs synchronize with the beat, facilitating the anticipation and processing of language over time [15, 28, 29]. Suppose you are learning a new dance, such as the ‘Cha Cha Slide,’ and an instructor is calling out rhythmic verbal instructions, such as ‘slide to the left,’ that help you to follow along. The rhythmic cue provided by the instructor helps your brain to anticipate and synchronize the action it has to perform — in this case, a dance — with brain waves in the temporal lobe, a brain region linked to rhythmic auditory processing [15, 28, 29]. While the rhythmic priming effect can result in a brief improvement in communication, more research is needed to determine how long the benefits to speech and grammar last [15].
Infants who are exposed to music every day, both with and without lyrics, demonstrate a better ability to recognize and differentiate speech than infants without exposure to music every day [17, 31]. Listening to music can improve recognition of human speech fundamental frequency [17]. Human speech fundamental frequency refers to the vocal cord vibrations that produce sounds, interpreted as pitches in the temporal lobe. Recognizing speech fundamental frequency helps a baby differentiate between the sound of their mother’s soft, soothing voice and the low rumble of a radiator [17]. Listening to music trains the temporal lobe to predict future auditory patterns more accurately, which may improve speech processing [29, 31]. An increased ability to understand and differentiate between sounds helps a baby to better process speech, in turn supporting early language acquisition [17].
Pediatric speech therapy can incorporate strategies from music therapy to foster language skills in children [7]. Young children’s brains are constantly developing as they are shaped by their interactions with the world around them [32]. As children learn, their brains exhibit neuroplasticity, a phenomenon that
describes the dynamic restructuring of neural connections over time [32, 33]. Since neuroplasticity decreases with age, music is most effective at aiding in language acquisition early in life [34]. Incorporating music into early speech therapy could be particularly beneficial for children with communication disorders [7, 32]. SLPs might help children play with toy instruments, an activity that requires imitation and activates mirror neurons [9, 35]. Mirror neurons are cells that activate when someone copies an action they observe another person performing [31]. For example, if you watch someone yawn, your mirror neurons may also cause you to feel the urge to yawn [31]. If a therapist plays a toy drum and encourages the child to copy the action, the child’s mirror neurons are activated to imitate the therapist’s actions [9, 35]. When mirror neurons are engaged and strengthened through music, children may find it easier to imitate speech they observe in others [9, 35]. Furthermore, the auditory-motor feedback from hitting a drum and hearing its sound helps reinforce the connection between listening and coordinating muscle movements. Auditory-motor feedback is a process needed for speech production [9, 35]. SLPs can also musically engage a child through singing together [7]. An SLP may use songs where the child fills in missing words or repeats the therapist’s words. Simple and repetitive songs such as ‘Row, Row, Row Your Boat’ are suitable for speech therapy because the children will hear the same sounds and syllables repeated again and again at a slow tempo, helping the children better understand how words are structured [7]. Through rhythm, repetition, and shared engagement, music can support processes necessary for speech production, from imitation to articulation [7, 36].
In addition to contributing to pediatric speech therapy, music can enhance language skills in adults [37]. Although adults exhibit less neuroplasticity than children, making it harder to learn new language skills, adults with communication disorders can also benefit from implementing music in speech therapy [10, 32]. When listening to your favorite song, you might feel happy, be tempted to dance, and even sing along. Without realizing it, you have used parts of your brain associated with emotion, small and large motor skills, speech, listening, and memory [37, 38]. Music helps adults with neurological damage build new networks of interconnected brain cells [37]. A person with damage to Broca’s area may struggle to produce speech, a condition known as non-fluent aphasia, but they often retain the ability to sing [10]. Music may strengthen the brain’s ability to find new neural networks to successfully produce speech without relying on the damaged Broca’s area, helping a person regain lost skills and facilitating remarkable recovery in those with non-fluent aphasia [10, 37].
Music can help adults who have suffered brain damage and lost language capabilities by gradually strengthening the relationship between rhythm and language [10, 11]. Melodic intonation therapy (MIT) involves a person copying syllables and words while tapping in sync to a rhythm and melody set by an SLP [10, 11]. MIT can improve the relationship between rhythm and language [10, 11]. Using a gradual approach consisting of steps that slowly increase in difficulty, MIT starts with simple syllables and slowly progresses to full sentences [10, 11, 39]. Together, regular rhythm and melody facilitate speech production by strengthening the neural pathways in and between both brain hemispheres, which is especially beneficial if one side has undergone significant damage [39]. A person with a communication disorder may initially learn to say, ‘I am hungry,’ and, ‘I need you to help me,’ with help from an SLP, and eventually, they will be able to speak in fuller sentences without needing to follow the therapist’s rhythm or melody [11, 39]. MIT is especially helpful for adults — who are more likely than children to experience a stroke, dementia, Parkinson’s disease, and traumatic brain injury — because it helps the brain create new pathways around the damage [10, 11, 39].
The use of music in speech therapy can enhance communication and self-advocacy skills for individuals with autism spectrum disorder (ASD) [40, 41]. ASD is a neurodevelopmental disorder characterized by repetitive behaviors and challenges with sensory regulation and social communication. Communication in individuals with ASD can range from nonverbal forms to verbal articulation [41, 42]. People with ASD face communication challenges because their brains have different levels of functional connectivity [42, 43, 44]. Functional connectivity (FC) is a metric that represents the dependent activation of one brain region on another [45]. Individuals with and without ASD have different levels of FC [42, 43, 44]. When FC deviates from the baseline level of an individual without ASD, speech deficits can occur [47]. Therefore, having both increased and decreased connectivity can be detrimental to an individual’s ability to communicate [42, 43, 47, 48]. For example, Broca’s area may struggle to communicate with a region associated with language comprehension called Wernicke’s area due to lower functional connectivity between regions [42, 43, 44].
SLPs can use music in therapy to help people with ASD communicate, advocate for themselves, and socially connect with others [42, 48, 49]. One approach SLPs can use to improve self-advocacy and communication is auditory-motor mapping training (AMMT) [48]. AMMT is based on MIT; however, during AMMT, an SLP taps electronic drums that are tuned to match the pitch of the word they are singing. The person with ASD then imitates their SLP by repeating the word and tapping the drum. AMMT synchronizes movement, hearing, and vocal skills: tapping the drum helps link the motor aspect of speaking with listening, which makes it easier to learn new words. Drumming, as used in AMMT, also encourages imitation skills, which can help activate mirror neurons [48]. Mirror neurons help every person, with or without ASD, understand emotions and intentions in conversation, processes that are often challenging for people with ASD [42, 50, 51]. Have you ever seen someone cry and subsequently felt their sadness yourself? Empathetic reactions to someone's emotions result from mirror neuron functioning, which encourages imitation [51]. People with ASD often struggle to detect and relate to the emotions of others as a result of reduced mirror neuron activity [42, 48, 52]. Social and emotional struggles can be a contributing factor to heightened
levels of anxiety and social isolation in individuals with ASD compared to those without ASD [42, 50]. Using music therapy to promote mirror neuron activity for individuals with ASD may improve empathy and emotional understanding by strengthening mirror neurons in emotional contexts [42, 48, 52].
Music therapy can also help individuals with ASD become more motivated to speak and improve their ability to self-advocate [42]. In addition to aiding speech production, music therapy can facilitate socialization by activating the ventral tegmental area (VTA), which is a region of the brain associated with promoting reward-driven behaviors [53]. Activating the VTA triggers the release of dopamine, a chemical messenger that enhances learning, motivation, and the feeling of reward when tasks are accomplished [53, 54]. For example, listening to your favorite song stimulates your VTA, which triggers the release of dopamine, reinforcing the behavior and making you more likely to repeat it [53]. People with ASD may experience atypical development of the VTA, causing less dopamine to be released during social interactions, and music may be helpful to attenuate this underrelease [53]. Improving communication skills through music therapy also allows individuals with ASD to better advocate for their needs and preferences [49]. Rather than focusing on altering the traits associated with ASD, the goal of facilitating speech development is to empower individuals by equipping them with the tools to self-advocate. The ability to assert their own rights is crucial for people with ASD to receive the support they need [49].
‘Finding the words I want to say is very hard, and my thoughts seem like they are blank,’ reports a person with Parkinson’s [55]. Parkinson’s, a neurological disorder characterized by slow movement, tremors, and cognitive decline, can cause communication impairments [11, 56]. Neurological damage to the motor cortex or other brain regions controlling respiration and articulation can lead to dysphonia and dysarthria [57, 58, 59]. Dysphonia causes a weakened and raspy vocal quality; dysarthria causes slurred and impaired speech [58, 59]. However, singing enhances the communication of people with Parkinson’s by supporting the neurological and physiological processes involved in speech [11, 13, 60]. Controlled vocal cord movement and respiration are essential for both speaking and singing, making singing a valuable tool for improving speech clarity, vocalizations, and facial
muscle movements [11]. Therefore, singing in speech therapy may improve the vocalizations of someone with Parkinson’s from disjointed, slurred words into intelligible, smooth speech [12, 58, 59].
The communication difficulties caused by Parkinson’s can become isolating for the individual [55]. ‘My husband has lost his initiative in social situations. He listens but rarely contributes,’ describes the partner of someone with Parkinson’s [55]. Group singing is a therapeutic approach that can be used to address the disconnect that Parkinson’s can cause, as it improves the motor skills required for communication and brings people together [12, 13]. Together, people sing familiar songs like ‘Happy Birthday’ and participate in breathing exercises, vocal warm-ups, and rhythmic clapping [12]. Group singing strengthens the brain’s control over respiration, the vocal cords, and other muscles involved in speech production [13, 60, 61]. Singing involves highly coordinated movements that occur in an organized, sequential order [13]. For example, a person first regulates breath intake before singing a note, then adjusts the pressure in their vocal cords to create different volumes and pitches [61]. After producing the note, the brain has the task of coordinating audio input and output [13]. The brain synchronizes the sounds it receives with the right muscle movements necessary to sing the correct notes. For instance, when singing in a group, individuals will listen to the pitches and timing of those around them to remain synchronized with the group. The connection between the auditory and motor regions is stronger during group singing than when playing an instrument [13]. Compared to speaking, singing demands more respiratory support, louder and more sustained vocalization, greater variation in pitch, and more accentuated articulation [13, 60]. Therefore, group singing practice effectively targets the motor challenges
faced by people with Parkinson’s. Many people with Parkinson’s struggle with dysphonia and dysarthria, so they benefit from the louder volume, improved clarity, and breath control promoted by group singing [13, 60]. Besides targeting motor skills, group singing also enhances neurological function [62]. Neural activation and connectivity increase when people sing, causing brain regions involved in movement and vocalization to become more active. Additionally, neuroplasticity heightens the temporal lobe’s ability to process auditory information, allowing singers to better modify their vocalizations [62].
The implementation of music into speech therapy is a transdisciplinary approach, strengthening speech therapy by incorporating methods from music therapy [7]. Language processing is not localized to a single area of the brain such as Broca’s area [12, 22, 35]. Instead, language processing is distributed across a vast network of regions [13, 22, 32]. Language involves auditory processing, coordinating the muscles used for speech, and using cognitive skills to understand speech and form words [13, 22, 32, 35]. Because language skills depend on many different regions of the brain, implementing music in speech therapy can foster neuroplasticity by building alternative pathways for communication between brain regions [13, 32, 33]. Music has benefits for people with a wide range of communication disorders, such as engaging social and linguistic development in people with ASD and supporting vocal strength in people with PD [12, 13, 42]. Despite the benefits of integrating music into speech therapy, there are still obstacles impeding the widespread adoption of music as a speech therapy practice [7]. Training SLPs to utilize new methodologies or collaborate with music therapists is expensive and difficult to achieve in low-income communities [7]. In order to promote the use of music in speech therapy, music as a treatment for communication disorders must continue to be studied [7]. Music has the potential to be a powerful tool in speech therapy and has already had a positive impact on many people with communication disorders [9, 10, 11]. However, we will only be able to fully measure its potentially life-changing impact on people with communication disorders once this treatment becomes accessible to everyone who could benefit from it [7, 10, 11].
References on page 94
by Thomas Doyle / art by Ava Sclafani
At some point in your life, you have probably felt blue, down in the dumps, or bummed out. To be depressed in the colloquial sense — generally sad or unhappy — is a familiar emotion, such that the English language provides us with plenty of words and phrases to describe the feeling. However, clinical depression is a mood disorder that goes beyond being in low spirits. Depression is characterized by a limited ability to feel pleasure, changes in appetite or sleep, low energy, diminished self-worth, and impaired concentration [1, 2]. The causes of depression vary from person to person and may entail psychological, social, and biological factors [3]. In recent years, one biological factor has been widely studied as a potential cause: neuroinflammation [4]. Inflammation is a bodily defense mechanism that protects the body
from infections, damaged cells, and toxins [5]. When inflammation occurs in the brain and spinal cord, it is called neuroinflammation [2]. While neuroinflammation is often protective, in some cases, it contributes to increased depressive symptoms [2]. Understanding the role of neuroinflammation in depression may help us gain further insight into biological contributions to depression as well as types of potential treatments [4].
THE BRAIN INFLAMED: WHAT IS NEUROINFLAMMATION?
Neuroinflammation is facilitated through the coordination of microglia and astrocytes [2]. Microglia and astrocytes are types of glial cells that are in the nervous system but are not neurons [6]. Glial cells
play an important role in supporting brain activity and providing an environment suitable for neurons to function [6]. Microglia maintain the conditions of their surroundings by performing regulatory functions, such as eliminating toxic substances [7]. Astrocytes carry out essential roles for proper neural functioning, such as regulating the brain’s breakdown of glucose to produce energy [8]. Additionally, astrocytes have branching extensions that radiate from their cell body, allowing them to interact with neurons at multiple distances, remove cellular debris, and respond to neural damage [9, 10]. The relationship between microglia and astrocytes in neuroinflammation resembles a jazz ensemble. By controlling the pace of the music, the pianist modulates the tempo of the entire group. Similarly, microglia regulate brain development, maintain neuronal networks, release signaling molecules to regulate cellular activity, and play a role in injury repair and immune response [2, 11]. Astrocytes mirror the ensemble’s trumpets, which support the other instruments by enriching the overall sound. Astrocytes support other cells’ functions by clearing debris and regulating the barrier between brain tissue and the blood [10].
Stressors in the brain, such as damaged tissues or viruses, trigger an immune response and induce neuroinflammation [2]. In the inflammatory response, microglia and astrocytes become more reactive to respond more effectively to stressors in the brain [12, 13]. Reactive microglia and astrocytes trigger increased cellular growth and reproduction [14, 15]. Reactive glial cells release cytokines, which are signaling molecules that influence the activity of recipient cells upon binding [13, 14, 15]. Pro-inflammatory cytokines increase the activity of recipient cells and increase levels of neuroinflammation, whereas anti-inflammatory cytokines decrease the activity of recipient cells and lower levels of neuroinflammation [13].
As the brain’s primary immune cells, microglia consume stressors in the brain to eliminate them from their environment [2, 11]. Reactive microglia can be classified as M1 and M2, while nonreactive microglia are labeled M0 [16, 17]. M1 and M2 microglia are not distinct subtypes — they exist on a continuum of states and can shift between them [16]. M1 microglia release pro-inflammatory cytokines, while M2 microglia release anti-inflammatory cytokines [16, 18].
Much like how M1 microglia increase neuroinflammation, the pianist raises the tempo, signaling the other musicians to follow suit. Conversely, in the same way that M2 microglia decrease neuroinflammation, the pianist slows down to signal other ensemble mem-
bers to lower the tempo. Similar to microglia, reactive astrocytes are categorized as either A1 or A2 astrocytes, with nonreactive astrocytes labeled as A0 [19]. A1 astrocytes tend to release pro-inflammatory cytokines to perpetuate neuroinflammation, whereas A2 astrocytes mostly release anti-inflammatory cytokines, dampening neuroinflammation [19]. Astrocytes increase their activity level as part of the immune response when they receive pro-inflammatory cytokines, like how a musician would increase their tempo when signaled by the pianist [20]. Reactive astrocytes form glial scars, increase their branching to surround damaged tissue, and direct repair cells to the area [21]. While glial scars can form barriers between damaged and healthy cells to limit the spread of neuroinflammation, they can also introduce problems by preventing cell growth [21, 22].
Properly regulated neuroinflammation facilitates the brain’s response to cellular damage by promoting the removal of injured or dysfunctional cells [23]. Removing damaged cells prevents unnecessary consumption of resources and ensures that materials are directed toward supporting healthy tissue repair and function [23]. Likewise, when the jazz ensemble’s
tempo is well-regulated, the music sounds harmonious, much like how properly-regulated neuroinflammation supports healthy brain function [23]. When the musicians are all playing at different speeds, the ensemble sounds chaotic and discordant. Similarly, when neuroinflammation is dysregulated, the microglia and astrocytes continue releasing pro-inflammatory cytokines, which leads to the damage and death of brain cells [2, 13, 14]. Dysregulated neuroinflammation may eventually result in psychiatric conditions like depression [24, 25].
Understanding neuroinflammation may help identify a neurological explanation for the changes in the brain that contribute to depression [25]. Reduced inflammatory activity in microglia and astrocytes is associated with a reduction in depressive symptoms [26]. Furthermore, reactive microglia have been shown to contribute to depressive behaviors by increasing the production of pro-inflammatory cytokines [26]. When neuroinflammation increases, astrocyte reactivity and depressive behaviors also increase [27]. The antibiotic minocycline has been found to inhibit the activation of microglia by blocking cytokine signaling that would typically lead to a reactive state [28]. In both rodents and humans, there is evidence that minocycline suppresses neuroinflammation, which in turn decreases
depressive behaviors [28, 29, 30]. Moreover, the drug scutellarin, traditionally used in Chinese herbal medicine, has been shown to suppress neuroinflammation and decrease levels of pro-inflammatory cytokines, also demonstrating a reduction in depressive behaviors [31, 32]. The use of minocycline and scutellarin in mitigating depressive behaviors implies that decreasing neuroinflammation could relieve depression [28, 31].
Since many neurological processes are similar between humans and rodents, animal models of depression can help researchers better understand the biological processes underlying depression and develop better treatments [33]. For example, minocycline was first tested in animal models prior to human applications [26, 27, 31]. Rodents have been a valuable tool for investigating the underlying biological mechanisms of depression [8, 26, 31]. While the brains of rodents and humans are structurally and functionally similar, there are distinct differences [34]. Human astrocytes, for instance, are larger than those of rodents and involve more complicated branching patterns [8]. Therefore, rodents serve as a model for human physiology and do not perfectly replicate human behavioral states, such as depression [8, 35]. Due to the limitations of animal models, research with human subjects is also important to translate results found in rodent studies to the treatment of human diseases [36].
Antidepressants are a standard form of depression treatment [37, 38]. However, they take time to become effective and can have negative side effects, including indigestion, sweating, and dry mouth [39]. Moreover, antidepressants are not guaranteed to effectively treat depression, leading individuals to turn to alternative forms of treatment [37, 38, 40, 41]. Transcranial magnetic stimulation (TMS) is one such treatment that could help improve our understanding of depression [42]. TMS uses a magnetic field to stimulate neurons in the outer layer of the brain, which has been shown to lessen symptoms of depression [42, 43]. People who underwent TMS treatment exhibited decreased levels of certain pro-inflammatory cytokines and demonstrated fewer depressive symptoms [43]. In addition, deep brain stimulation (DBS) could contribute to our understanding of depression in humans as well. DBS uses electricity to stimulate brain areas associated with depressive symptoms and alter their activity [42]. DBS is a promising therapy for
treatment-resistant depression; however, its impact on neuroinflammation is in the early stages and has yielded inconsistent findings [42, 44]. In some cases, DBS has been able to reduce neuroinflammation by increasing levels of anti-inflammatory cytokines in conditions such as epilepsy and traumatic brain injury [42, 45, 46]. However, other studies have reported increases in the levels of pro-inflammatory cytokines after DBS treatment, suggesting that the treatment leads to increased neuroinflammation [42, 47]. The variability in findings related to the efficacy of DBS may be a result of the treatment decreasing amounts of some pro-inflammatory cytokines while not affecting other cytokines [42]. More research is needed to better understand how TMS and DBS can reduce neuroinflammation to alleviate depressive symptoms [42].
Depression is a widespread and debilitating condition [3]. Just one type of depression, major depressive disorder, affects over 300 million people and is the most prevalent cause of disability worldwide [1, 48]. The causes of depression are poorly understood,
and standard treatments like antidepressants do not always relieve symptoms [48]. As a result, new treatments are needed to reach a greater proportion of the population with the condition [48]. While drugs such as minocycline and scutellarin decrease neuroinflammation and depressive behaviors in rodents, little is known about their effect in humans [28, 31].
Treatments like TMS and DBS have been administered to humans, but findings have been inconsistent as to whether they reduce neuroinflammation [42]. Gaining a more concrete understanding of the link between glial cells, neuroinflammation, and depression could help further develop TMS, DBS, and other treatments for depression, helping countless people [4, 42].
References on page 97
by Anoushka Bhatt / art by Nancy Duer
Pain is a distinctly personal experience; it is internal, often difficult to articulate, and largely invisible to the naked eye. Others can’t see it, touch it, or quantify it in a way that captures its subjective experience. The invisibility can be isolating. Even in the presence of support, people in pain can be left feeling alone and ostracized. For some people of color, these experiences are exacerbated by a medical system that not only fails to see their pain but questions whether their discomfort exists at all [1]. When suffering is dismissed and voices are silenced, it’s more than neglect — it is an institutional betrayal of care [2]. This isn’t just an individual experience; it is foundational to a long history of racial bias in pain medicine that continues to shape outcomes today [3, 4, 5]. In the early nineteenth century, scientists, physicians,
and slaveholders propagated myths about Black pain to justify slavery, one being that Black people’s skin was less sensitive to pain than that of White people [1, 6]. The medical mistreatment of Black people continued through the late 1800s, with notable figures in medicine like Dr. J. Marion Sims, known as the father of gynecology, conducting unethical medical experiments on enslaved Black women without anesthesia [6, 7, 8]. Similar maltreatment and harmful beliefs persist in the modern era of medicine [1, 9]. As recently as 2016, 50% of a group of White medical students and residents surveyed at a large public university held at least one false belief about pain in Black people [1]. Some fallacious beliefs included the notions that Black people’s skin was thicker, their nerve endings were less sensitive, and their blood clotted quicker than White people’s [1]. Historical injustices and misconceptions about pain have led to lasting maltreatment of people of color by medical institutions [1]. Medical bias is still entrenched in medical institutions and continues to affect the treatment of people of color today [10].
A multitude of barriers hinder people of color from accessing timely and effective treatment [11, 12, 13]. People of color face discriminatory obstacles originating from systemic racism, a term that refers to racial biases that are deeply entrenched within policies and practices [14]. Racial biases extend across interconnected systems, including education, housing, politics, criminal justice, and healthcare, all of which together perpetuate a lower standard of care for racial minorities [14] As a result, untreated and mismanaged conditions contribute to increased physical pain, heightened emotional distress, and diminished quality of life for many people of color [15, 16, 17]. Furthermore, racial minorities are more likely to live in areas that lack access to essential healthcare resources, known as healthcare deserts [12, 13, 18]. Individuals living in healthcare deserts face a lack of access to medical facilities, longer wait times, and disproportionately higher costs of health services [11, 13, 19]. Healthcare deserts pose a considerable challenge for communities of color to access even the
most basic healthcare needs, including primary care services, emergency medical services, pharmacies, and preventive medications [11, 19, 20].
A lack of access to healthcare not only limits general healthcare services but also hinders the diagnostic process of more severe diseases [21]. People of color face barriers to receiving essential preventive screenings — a crucial step in diagnosis [22, 23, 24]. When access to preventive screenings is impeded, diseases may progress unchecked, symptoms and pain can be exacerbated, and the risk of fatal outcomes may increase [21]. For example, Indigenous American people face significantly lower cancer screening rates despite having the highest risk and mortality rate for various cancers, including kidney, liver, stomach, and cervical cancer [25, 26, 27]. A lack of early detection can manifest in delayed diagnoses and advanced disease stages, potentially contributing to additional pain and the need for more invasive treatments [28, 29, 30]. Indigenous women exhibit the lowest rates of mammography, a form of X-ray imaging designed to detect cancers and other changes in breast tissue [25]. Only 59% of Indigenous women receive mammograms, compared to 76% of White women who are screened for breast cancer [25, 31]. Moreover, Indigenous women experience a higher likelihood of suffering from late-stage cancer complications and have a 30% higher breast cancer mortality rate than White women [25, 31]. Later-stage breast cancer can be excruciatingly painful as the cancer spreads throughout the body and invades nerves and bone [32]. Breast cancer that infiltrates the nerves can result in agonizing, sharp, burning pain that radiates through the body, while cancer invading the bone can cause a deep, aching pain [33, 34, 35]. Furthermore,
chronic inflammation associated with breast cancer triggers hypersensitivity in the nervous system, leading to heightened, persistent, and widespread pain that extends beyond the tumor site [36, 37, 38]. In the advanced stages of breast cancer, pain becomes more widespread and often requires more invasive interventions for relief [39]. Lower rates of breast cancer screenings and diagnoses can lead to delayed intervention, resulting in more painful cases of the disease among Indigenous women [26, 27]. Systemic barriers that limit access to preventive care contribute to a cycle of pain that disproportionately burdens communities of color [15, 40, 41].
Even for those who receive preventive testing, racial biases continue to disproportionately affect healthcare outcomes through diagnostic tools and algorithms like risk prediction models and machine-learning systems [42, 43, 44]. Medical algorithms, such as those used to calculate stroke risk or predict kidney failure, offer a framework to help healthcare professionals make informed decisions based on an individual’s clinical data [45, 46, 47]. However, not all medical algorithms can be generalized to all races, as different ethnic groups can have genetic and biological differences that may result in the disproportionate occurrence of certain conditions across groups [48]. Thus, generalized references provided by these algorithms are often unsuited to assess people of color [43, 49]. Reference intervals are a key component of medical algorithms, which are sets of values defining the standard range for a given test result [50]. That being said, reference intervals tend to be developed utilizing data from medical studies primarily consisting of White male participants, leading to biased assessments for other racial and gender groups [43, 49, 51]. Over 50% of laboratory tests with reference intervals fail to account for differences in baselines between racial groups, revealing a major representational issue in categorizing diagnostic results [52].
A notable example of an algorithm generalized to people of color is the atherosclerotic cardiovascular disease (ASCVD) risk calculator, which estimates a person’s ten-year risk of heart disease or stroke [53, 54]. The ASCVD calculator factors in age, cholesterol levels, blood pressure, and race — categorizing individuals as White, Black, or ‘other,’ with an option to exclude race entirely from the calculation [53, 54]. However, the limited racial categories fail to distinguish between diverse populations within the ‘other’ group, making it difficult to account for critical
variations such as age-related risk and cholesterol levels [55]. Compared to White populations, South Asian populations tend to develop cardiovascular disease at a younger age and have a higher prevalence of heart attacks and strokes [56, 57]. South Asian people also tend to experience more severe disease outcomes at lower cholesterol levels compared to other racial groups, suggesting that traditional reference ranges may not be reliable for assessing cardiovascular risk in this population [57, 58, 59]. Therefore, the ASCVD risk calculator fails to account for unique risk factors that contribute to the underdiagnosis and undertreatment of cardiovascular conditions in South Asian individuals [58, 60]. As a result of undiagnosed ASCVD, South Asian people may endure prolonged chest pain, fatigue, and other debilitating symptoms before receiving an accurate diagnosis [61, 62]. ASCVD can also manifest in neck, back, and even abdominal pain when unmanaged [61, 62]. As risk factors accumulate and progress to heart disease, pain may spread further to the arms or legs, often accompanied by shortness of breath, palpitations, nausea, sweating, or lightheadedness [62]. Beyond cardiovascular disease, the broader implications of biased medical algorithms extend to many other conditions, including kidney disease and vitamin B12 deficiency, exacerbating racial discrepancies in pain management [44, 52, 50]. Racial minorities are more likely to have their pain underestimated and undertreated due to biases in diagnostic tools, contributing to unnecessary suffering and, consequently, lower quality of life [1, 63]. Individualized approaches incorporating
genetics, lifestyle, and socioeconomic factors could improve the accuracy and equity of cardiovascular risk assessment [64]. By addressing biases in medical algorithms and ensuring that diverse populations are adequately represented in research, healthcare systems can provide more precise diagnoses and effective symptom management that would ultimately improve pain outcomes across racial groups.
Upon immediate arrival at emergency rooms, people of color face further inequities in pain assessment. [65, 66, 67]. Black people experience 35% longer wait times than White people afflicted with similar conditions, delaying access to critical pain management and medical intervention[66, 68, 69]. Delayed care for Black, Asian, and Hispanic people significantly reduces their chances of receiving timely interventions for potentially life-threatening strokes and often results in prolonged, unmanaged pain [69]. When analyzing an individual’s condition to determine how quickly the person receives treatment — otherwise known as an urgency evaluation — a healthcare provider would ideally base their assessment solely on medical urgency [70]. However, urgency evaluations can be distorted by implicit biases that minimize or dismiss the pain experiences of people of color [70]. The longstanding and harmful myth that people of color have a higher pain tolerance contributes to the systematic underestimation of their suffering, leading to the dismissal of both self-reported pain and even physically evident distress [1, 71, 72]. As a result of discrepancies in assessment, people of color may be assigned lower priorities for receiving care despite presenting with severe symptoms [70, 73, 74]. Lower-priority assignments in healthcare can manifest as longer wait times, reduced attention from medical professionals, and the dismissal of individual concerns [74]. Disparities in access to adequate and timely care not only aggravate immediate suffering but also lead to longterm health consequences, reinforcing a cycle of pain mismanagement and eroding trust in the healthcare system [75, 76].
Once admitted to the hospital, inequities in pain assessment can quickly lead to discrepancies in pain management for people of color [1]. Black people are significantly less likely to receive adequate pain medication than White people, even for identical pain reports [1, 77, 78]. Due to stereotypes about people of color’s drug-seeking behavior, which was partly informed by the opioid crisis, racial minorities are less likely to be prescribed opioids, a powerful and
addictive class of pain medication [79, 80]. The opioid crisis was at first characterized by a medical overprescription of opioid drugs [81, 82]. Historically, the crisis was concentrated among White, rural, and working-class communities [79, 83]. However, the impact of the opioid crisis on Black and Hispanic communities has worsened substantially in recent years, influencing stereotypes about opioid use within these communities [79, 83]. As extensive opioid abuse worsened and public health efforts sought to curb overprescription, the rise of fentanyl — a synthetic opioid that is often added to illicit substances — shifted the influence of the crisis from white communities to communities of color [81, 84]. Greater use of illegal drugs laced with fentanyl within Black and Hispanic communities led to a disproportionate rate of opioid overdose and addiction, shaping harmful stereotypes about drug-seeking behavior in medical settings [79, 83, 85]. These stereotypes about drug-seeking behavior contribute to the underprescription of opioids in people of color compared to White people [79, 80]. Even when opioids are prescribed, Black and Hispanic people receive lower dosages than White people for equal levels of self-reported pain as a result of drug-seeking stereotypes [79, 85]. Underprescription may contribute to individuals seeking pain relief from illicit substances beyond prescription medications [87, 88]. Not only do White people have better access to opioids to manage their pain, but they are also more likely to be shifted to alternative pain management solutions in situations of opioid dependence or addiction [80, 83, 89]. Black people, on the other hand, are often denied opioids altogether and are much less likely to receive nonopioid forms of pain treatment, like nerve blocks, physical therapy, and surgery [80]. Limited access to alternative treatments may contribute to the dual burden faced by Black communities, being both underserved in pain treatment and criminalized for opioid use rather than being offered rehabilitation approaches [83]. The long-term effects of prescription and rehabilitation disparities continue to shape healthcare experiences, reinforcing mistrust in the medical system and leaving many Black people without adequate pain relief [83, 90, 91].
Racial differences in pain treatment also extend to vulnerable individuals within minority populations, such as children and pregnant people, whose pain is frequently dismissed [92, 93, 94]. For instance, Black and Hispanic pregnant people frequently report having their pain dismissed or undertreated, both during childbirth and in the pre- and post-partum periods [93, 94]. Disregard for the pain of Black and
Hispanic pregnant individuals contributes to disproportionately high rates of maternal complications in these communities [93, 95]. Children of color are also affected by racial disparities in medicine; for example, Black children experiencing appendicitis are far less likely to receive appropriate pain relief compared to White children [92]. Asymmetry in pain treatment is also evident in the treatment of chronic illnesses like type 1 diabetes, where access to effective treatment can significantly reduce disease progression and prevent complications such as nerve damage [96]. Black and Hispanic children are less likely to have access to advanced diabetes management technologies, such as continuous glucose monitors and insulin pumps, which can significantly improve long-term health outcomes [97]. Perceived bias and discrimination, poor patient-provider communication, unequal treatment outcomes, and broader systemic barriers disproportionately affect Black and Hispanic children, contributing to differences in care [98]. Persistent disparities in pain assessment and treatment reflect deeply rooted systemic inequities that continue to harm the most vulnerable members of minority communities [99, 100, 101].
The consequences of inadequate pain treatment are profound and long-lasting [102, 103, 104]. Unmanaged pain can have severe long-term consequences due to its profound impact on the nervous system and overall well-being [102, 103, 104]. When pain persists without proper management, the nervous system becomes hypersensitive, amplifying pain signals to make even mild discomfort feel unbearable [105, 106]. Over time, pain pathways in the brain are reinforced by maladaptive neuroplasticity, a process that essentially ‘rewires’ the nervous system to prioritize pain perception [102, 107]. Without early intervention, changes in neurological pain perception pathways can entrench individuals in a cycle of escalating pain, emotional distress, and functional impairment, making chronic pain progressively more difficult to manage and treat [108]. This neurological rewiring does not occur in isolation; it often coexists with psychological responses like anxiety and depression, which further reinforce the pain cycle [109, 110, 111]. As a result, people who suffer from chronic pain due to inadequate treatment often experience diminished quality of life and struggle to complete daily activities [112]. For communities already facing barriers to care, these long-term consequences contribute to broader health disparities and place an additional burden on public health systems [22].
Furthermore, the persistent dismissal of pain concerns can foster medical distrust and discourage people of color from seeking care altogether [10, 91, 113]. Communities with histories of medical exploitation, such as the Black community, continue to feel the effects of unethical studies [114]. In the infamous Tuskegee Syphilis Study, hundreds of uninformed Black men were studied to examine the effects of syphilis when left untreated [114]. While informed consent was not legally required when the study began in 1932, the Tuskegee Syphilis Study was unethical even by the standards of its time [115]. Participants were not told they had syphilis but instead that they had ‘bad blood’ to conceal the true purpose of the study and to recruit more participants [116]. Despite penicillin becoming a widely available cure for syphilis during the study, it was never offered to any of the affected men [114]. The participants were also not informed that medication was intentionally withheld from them or that treatment was even available [114]. As a result of the medical malpractice by
the doctors and researchers, these men had to endure the symptoms of untreated syphilis [114]. Those infected with syphilis are subjected to a lifetime of suffering, beginning with sores and progressing to symptoms as extreme as bone and joint damage, cardiovascular problems, hearing loss, and blindness [117]. The impact of the Tuskegee Syphilis Study was not limited only to the participants; due to the highly transmissible nature of syphilis, the spouses and children of the men studied were at higher risk of becoming infected and ill [118]. The unethical deception and neglect displayed in the Tuskegee Syphilis Study reinforced a mindset of medical mistrust that persists today within the Black community [114, 116, 119]. Coupled with the ongoing disparities in pain management and treatment, historical trauma contributes to reluctance in seeking medical care and further exacerbates health inequities [114]. The legacy of unethical practices continues to shape perceptions of the healthcare system, fostering skepticism and fear that prevent Black individuals and other minority groups
Forced sterilizations of women of color under oppressive and coercive medical policies further deepened medical mistrust in people of color [120, 121, 122]. In the early to mid-20th century, the U.S. government and medical institutions forcibly sterilized thousands of Black women, particularly in the South [120, 122]. The sterilization was based on eugenics, an ideology centered around the selective breeding of people based on prejudiced, ableist, and often racist perceptions of positive or negative traits [120, 122]. Harmful beliefs popularized by eugenic rhetoric falsely deemed thousands of Black women unfit for reproduction [120, 122]. Similarly, Indigenous women were disproportionately targeted for sterilization in the 1960s and 1970s, with many undergoing the
procedure without their consent or true understanding of its permanence [123]. Latina women also suffered this violation, as seen in the 1978 Madrigal v. Quilligan case, where many Mexican-American women were coerced into sterilization procedures, often during childbirth [121]. Racist and unethical medical practices such as these sterilizations were rooted in population control efforts and have had lasting effects on minority communities, fostering profound and persistent mistrust in the current healthcare system [120, 121, 123]. The traumatic legacy of forced sterilizations continues to shape the experiences of women of color within the healthcare system today, deterring them from seeking reproductive care due to fears of bodily autonomy violations [124, 125]. The historical exploitation of marginalized communities in the name of medical progress continues to fuel skepticism of healthcare systems, making it imperative to address injustices to rebuild trust and ensure equitable healthcare for all [126].
Ultimately, the racism embedded in pain assessment and treatment is not an isolated issue but is symptomatic of a much larger, interconnected web of systemic oppression [5, 14]. Just as discriminatory practices in healthcare have long-lasting repercussions on the physical and emotional well-being of marginalized communities, systemic inequalities in housing, education, employment, and the criminal justice system also reinforce and perpetuate racial disparities [14, 22]. These systems do not operate in isolation; they intersect to create compounded disadvantages that disproportionately impact people of color [14]. The dismissal of pain, violation of bodily autonomy, and historical exploitation of people of color are all reflective of a broader societal structure that continues to devalue marginalized lives [127]. Recognizing medical racism as a public health threat that manifests in both physical and emotional pain can begin to rebuild trust, promote equity, and ensure that healthcare operates justly and humanely for everyone [128].
References on page 100
by Daniel Wunschel | art by JD Jaromilek
You have a seemingly endless list of work to complete: an essay to write, a presentation to make, and a book to read, but you haven't even started. Lying in bed all day, you hope your work will get done with no physical effort. But what if you could write your essay, complete your presentation, and read your book by just thinking about it? You stare at the ceiling and think about turning on the lights, and suddenly, your room brightens. On your way to the kitchen, you think about turning on the coffee machine, which subsequently stirs to life. With a cup of coffee in hand, you sit down, open your laptop, and begin to think about writing your essay as the words you envision fill the screen. While this may seem like a world of science fiction, certain biomedical companies are attempting to bring these ideas closer to reality by developing brain-computer interfaces (BCIs). BCIs are hardware and software systems that capture the user’s brain activity and translate it into commands that can control devices such as a computer cursor, robotic limb, or electric wheelchair [1, 2]. Neuralink is a company that intends to create a commercialized, surgically implanted BCI that can translate thoughts into actions [3]. The main stated goals of the company are to use their device to restore mobility in paralyzed individuals via controlled prosthetics, improve communication for non-verbal people, treat neurological conditions, and enhance cognitive functions such as memory. But while Neuralink’s proposal sounds revolutionary, the mechanisms of Neuralink’s technology are ambiguous and pose substantial ethical issues [3, 4].
BCIs work by measuring the electrical activity of neurons in the brain [2]. Neurons play a crucial role in communication within the brain and can either be on, actively transmitting signals, or off, at rest and transmitting no signals [5]. While at rest, neurons maintain a constant voltage known as the resting membrane potential [6]. The voltage of a membrane is determined by the concentration of charged particles, called ions,
inside the neuron compared to the ion concentration directly outside the neuron. Neurons generally maintain a negative resting potential, meaning there are more positively charged ions outside the neuron than inside. When a neuron receives a signal from another neuron, channels open that allow ions to flow in and out of the neuron, changing the neuron’s voltage. The flow of many positive ions into the neuron can trigger an action potential, a rapid increase in the cell's voltage that allows for communication between neurons. Every bodily function or activity results in specific neurons firing action potentials, which a sensor can record as ‘spikes’ to create a unique pattern [6, 7]. For example, moving your fingers to type on a keyboard generates a unique set of spikes [7]. Interestingly, performing an action and thinking about performing that same action produces comparable spike patterns [8]. BCIs record these spikes and convert the data into information that an external computer can process [9]. The external computer can then control connected devices, such as prosthetics, based on the specific spikes [9].
A multi-step process allows BCIs to record spikes and subsequently control devices [10]. As an example, someone who is paralyzed in all four limbs and would like to use a BCI to control their wheelchair would first have their brain activity recorded to determine the spiking pattern associated with wheelchair movement. Most BCIs record spikes using techniques such as electroencephalography (EEG), which monitors neuronal electrical activity via sensors placed on the scalp [2, 10]. The EEG would record the activity as the person imagines rolling their wheelchair back and forth, resulting in a spike pattern. Since any and all movements generate spikes, subtle actions like blinking or coughing create irrelevant signals — known as ‘noise’ — which have to be filtered out to focus on the activity of interest [11]. Artificial intelligence software helps to discard and filter out the noise and extract the relevant signal to be analyzed [10]. Next, processing algorithms pair the spikes to their corresponding action. The BCI’s refinement of
data can be likened to editing an essay; you start with a rough draft, then remove any unnecessary words or phrases to clarify meaning, culminating in a final essay that is ready to submit. Once refined, the data would be sent from the connected computer to the wheelchair, activating a motor to move the wheelchair in the desired direction [2, 10, 12].
Since spike patterns are unique to each action and person, BCIs must be calibrated specifically to the user [13, 14]. Calibration typically involves exercises such as moving an on-screen cursor, moving one’s arm, or merely imagining such movements, allowing the BCI to recognize their specific spike patterns and link them to corresponding actions [14]. The calibration process can be taxing for users, as sessions can last between 15 to 30 minutes, involve a varying number of activities depending on the user, and may require up to 80 trials per activity [15]. While BCIs aim to assist individuals with disabilities, for some people, the calibration process can ultimately detract from their treatment rather than aid it due to the mental energy required [16]. Despite their drawbacks, BCIs
have successfully enabled people to control cursors, robotic arms, and wheelchairs, offering greater independence and improved quality of life for those with disabilities [17].
Most BCIs are non-invasive, meaning they are not surgically implanted into the brain [18]. In contrast, Neuralink claims that their device, ‘the Link,’ detects spikes more accurately than other BCIs because it is directly implanted in the brain [19]. Non-invasive EEG-based devices are less sensitive than invasive EEGs, such as the Link, since they sit on the scalp and are physically farther from the neurons generating the spikes [20]. Distance from recorded neurons may make non-invasive devices more prone to contamination of noise, such as electrical signals from the body and even household electronics [21]. Neuralink claims that their device detects spikes more accurately than EEG-based BCIs because of the Link’s direct implantation in the brain [22].
Specifically, the Link is implanted into the sensorimotor cortex — the brain region responsible for controlling movement — so that the device can precisely record spikes related to movement in individuals using the device for motor disabilities or needs [22, 23]. The Link is designed to achieve high precision by inserting an intricate system of threads into the brain [24]. In comparison to the rigid threads found in older BCIs, Neuralink’s threads are upgraded to be flexible, microscopic strands of synthetic material that contain electrodes. The flexible material in the Link’s threads allows them to move with the brain, which prevents damage to the surrounding brain tissue [25]. The electrodes contained in these threads are delicate sensors that record neuronal activity [22, 24]. The invasive design of the Link also allows the implant to record groups of neurons [20]. When
Neuralink's threads are implanted, they are placed on a region of interest, such as specific neurons in the sensorimotor cortex [26]. By placing threads directly on top of certain neural regions, the electrodes can record signals from distinct populations and avoid picking up on background noise that EEG-based BCIs encounter. Neuralink can then record voltage changes in targeted neuronal groups and detect the activity of those neurons with higher clarity [20]. Neuralink’s invasive approach enables highly accurate spike recording, which may lead to clearer signals being sent to connected devices [27].
Another important factor that theoretically enhances the Link’s precision is the large number of sensors that the device uses [26]. The Link is equipped with 32 threads, each containing 96 electrodes, totaling 3,072 electrodes [26]. Neuralink’s work reflects a significant increase in electrodes compared to previous BCIs, which had no more than 256 electrodes [28]. A higher electrode count allows for greater precision by detecting more spikes, ultimately improving the device’s functionality [22]. Past BCIs were limited by the difficult and time-consuming process of manually implanting numerous threads into the brain by hand [22]. Neuralink has circumvented such technical constraints by developing a robotic system to insert the threads [26, 29]. Automation of the implantation process makes it possible to insert more electrodes precisely, thus improving the Link’s spike detection and, ultimately, its functionality [22].
While Neuralink's technology already presents significant strides in technological development, the company aims to create a new type of BCI that would restore mobility in users [22]. The Link currently functions as a read-out BCI, meaning it receives and records neural signals that are often used to control a device, as with most BCIs [30]. In comparison, writein BCIs contain devices that send signals to neural tissue. However, Neuralink’s future goal to eventually restore mobility in users would likely cause the device to act as both a write-in and read-out BCI. Write-in BCIs are widely used as therapeutic devices that work by stimulating the neural tissue in certain brain regions with electricity or light. Cochlear implants are a type of write-in BCI device that functions therapeutically in the ear. A cochlear implant restores some auditory function in individuals with hearing impairments by stimulating auditory nerves [30]. Methods similar to the one utilized by the cochlear implant could theoretically be applied to other losses of function, like movement or vision, by utilizing the Link as a write-in BCI [5, 31].
Neuralink has set ambitious goals but has not yet published a practical explanation of how its goals will be achieved [32, 33]. Although the Link offers a functional replacement for movement, the company also claims that its technology could restore mobility in paralyzed individuals [24]. However, Neuralink does not specify how the technology will accomplish this or acknowledge potential limitations to their goal. For example, injuries such as spinal cord trauma may impede neurons’ ability to successfully generate action potentials, which would hinder the BCI’s effectiveness in restoring mobility. If neurons cannot generate action potentials, the Link would be unable to detect spikes and thus be unable to control an external device. While Neuralink may plan to restore mobility in paralyzed individuals, their technology cannot currently regenerate activity in degraded neurons [24].
In addition to restoring mobility, Neuralink aims to use the Link for nerve and brain stimulation to treat conditions like blindness [5, 31]. Electrically stimulating parts of the visual pathway — such as the
retina, optic nerve, thalamus, or visual cortex — may aid in restoring aspects of visual perception. However, because vision processing relies on complex networks across multiple brain regions that develop over time, the efficacy of electrical stimulation is yet to be assessed [5, 31]. Similarly, electrical stimulation of motor-related brain regions may potentially facilitate movement through the reactivation of dormant motor pathways, though such treatment is currently unstudied [30, 34]. While stimulation-related advancements seem promising, the long-term effects of neural stimulation are largely unknown [25, 30, 34]. The Link is a permanent implant, so if it loses functionality after being implanted, one would need to undergo a second surgery to have it removed [36]. However, the removal process carries its own risks. Surrounding tissue that adheres to the device would need to be removed along with the implant, which could potentially cause brain tissue damage and carries a great risk of infection [36]. Since the long-term effects of invasive BCIs are not publicly available or even well-studied, is it worth the surgical and health risks associated with the procedure to get a device that has uncertain durability and longevity [37]?
Moreover, despite Neuralink’s claims of starting human trials with three participants, they have not yet published any supporting data in a peer-reviewed scientific journal [32, 33]. Sharing scientific research is crucial to the advancement of science, as it allows others to review and replicate findings as well as conduct further studies [38]. The only peer-reviewed publication — as evaluated by qualified members outside of the original research group — from Neuralink thus far is based on animal experiments [26]. Animal models can be useful in predicting outcomes in humans, but the results are not directly translatable [39]. The lack of peer-reviewed publications makes it difficult for the scientific community to assess the validity of Neuralink’s claims [32, 33]. Someone interested in using the Link may be unable to find trustworthy, peer-reviewed information to corroborate the data on Neuralink’s website [32, 33]. Additionally, the U.S. Food and Drug Administration (FDA) initially rejected Neuralink’s device in 2022, citing concerns about the device’s implantation, potential thread migration to other parts of the brain, and device removal [40]. While the device is now approved for clinical trials, it is unclear what changes were made to address the FDA’s concerns, as the company has not publicly disclosed this information [40].
In addition to the technological limitations of Neuralink, the company’s ambitious goals are constrained by ethical concerns. One key concern is the protection of personal data [41]. As data privacy becomes increasingly important in safeguarding personal information, protecting neuroscience data — data related to brain activity — has become crucial. Currently, neuroscience data is used to create large datasets that may enable new insights into brain function in both healthy and diseased states. However, as with other types of personal data, issues of privacy arise. Accessing or analyzing neuroscience datasets that contain imaging data, such as those used by Neuralink, may reveal an individual’s cognitive and emotional states, such as moods, feelings, and thought patterns. Furthermore, analysis of neuroscience datasets may be used to predict an individual’s future outcomes, such as the likelihood of developing neurological disorders or even the potential risk of criminal behavior, which may lead to the unwanted disclosure of personal information [41]. Since BCIs record and potentially store neuroscience data, one’s data could be accessed and analyzed by various companies or researchers [42]. Despite the personal nature of neuroscience data, there are relatively few regulations and laws regarding how the data is shared, stored, and used [41, 43]. For example, the California Consumer Privacy Act [44] provides regulations for biometric data, such as fingerprints and DNA, but does not clarify whether neuroscience data is a part of this category [43]. While the CCPA restricts the use of biometric data for identification purposes, it does not impose restrictions on its use to infer an individual’s mental state [43]. The aforementioned example highlights the need for clearer regulations that distinguish neuroscience data from other forms of biometric data and limit how neural data can be utilized, particularly by large corporations.
By translating thought into action, BCIs allow people to regain a connection between brain and body, and the Link is one of many BCIs contributing to this restoration [37]. However, unlike other BCIs, the Link’s unique methods and ambitious goals raise serious scientific and ethical concerns [24, 41]. The Link’s potential to revolutionize treatments for various neurological disorders, such as paralysis, could significantly improve the quality of life for millions [24]. However, the complexity of the technology and the risks associated with invasive brain surgery, such as infection
or brain damage, cannot be overlooked [37]. Moreover, the company has yet to produce peer-reviewed literature demonstrating their results, making it difficult for others to assess the validity of their claims through reexamination and replication [32, 33]. The lack of published research and adequate regulatory oversight raises serious concerns regarding the efficacy of Neuralink [32, 33]. Thus far, Neuralink’s sample size is very small, consisting of only three individuals who have received the implant [45, 46]. The lack of verified information surrounding the technology may discourage additional participants [33]. Such a limited sample size makes it difficult to generalize the results and draw definite conclusions to further progress toward the commercialized use the company envisions [47]. While the promise of Neuralink is compelling, it requires much more rigorous testing, thoughtful regulation, and broader societal discussion before it can be deemed safe and beneficial for widespread use [24, 48].
References on page 107
1. Hyde, J. S., Bigler, R. S., Joel, D., Tate, C. C., & van Anders, S. M. (2019). The future of sex and gender in psychology: Five challenges to the gender binary. American Psychologist, 74(2), 171–193. doi:10.1037/amp0000307
2. World Health Organization. (n.d.). Gender. Retrieved April 12, 2025, from https://www.who.int/ health-topics/gender#tab=tab_1
3. American Psychiatric Association. (2022). Gender dysphoria. In: Diagnostic and Statistical Manual of Mental Disorders (5th ed., text rev.). American Psychiatric Publishing. doi:10.1176/appi. books.9780890425787.x14_Gender_Dysphoria
4. Kaufman, M. R., Eschliman, E. L., & Sanchez Karver, T. (2023). Differentiating sex and gender in health research to achieve gender equity. Bulletin of the World Health Organization, 101(10), 666–671. doi:10.2471/BLT.22.289310
5. World Health Organization. (2025). ICD-11 for mortality and morbidity statistics: HA60 gender incongruence of adolescence or adulthood. Retrieved April 12, 2025, from https://icd.who.int/ browse/2025-01/mms/en#411470068
6. Claahsen-van der Grinten, H., Verhaak, C., Steensma, T., Middelberg, T., Roeffen, J., & Klink, D. (2021). Gender incongruence and gender dysphoria in childhood and adolescence—Current insights in diagnostics, management, and follow-up. European Journal of Pediatrics, 180(5), 1349–1357. doi:10.1007/s00431-020-03906-y
7. Hembree, W. C., Cohen-Kettenis, P. T., Gooren, L., Hannema, S. E., Meyer, W. J., Murad, M. H., Rosenthal, S. M., Safer, J. D., Tangpricha, V., & T’Sjoen, G. G. (2017). Endocrine treatment of gender-dysphoric/gender-incongruent persons: An Endocrine Society clinical practice guideline. The Journal of Clinical Endocrinology & Metabolism, 102(11), 3869–3903. doi:10.1210/jc.201701658
8. Chaku, N., & Barry, K. K. (2023). Exploring profiles of hormone exposure: Associations with cognition in a population-based cohort of early adolescents. Infant and Child Development, 32(3), e2415. doi:10.1002/icd.2415
9. Gurvich, C., Hoy, K., Thomas, N., & Kulkarni, J. (2018). Sex differences and the influence of sex hormones on cognition through adulthood and the aging process. Brain Sciences, 8(9), 163. doi:10.3390/brainsci8090163
10. Schwartz, E., & Ketner Villa, J. (2024). Role of hormones over the lifespan: How hormone balance affects general health and well-being at all ages. Women’s Health Problems – A Global Perspective. doi:10.5772/intechopen.114213
11. Coleman, E., Radix, A. E., Bouman, W. P., Brown, G. R., de Vries, A. L. C., Deutsch, M. B., Ettner, R., Fraser, L., Goodman, M., Green, J., Hancock, A. B., Johnson, T. W., Karasic, D. H., Knudson, G. A., Leibowitz, S. F., Meyer-Bahlburg, H. F. L., Monstrey, S. J., Motmans, J., Nahata, L., Nieder, T. O., Reisner, S. L., Richards, C., Schechter, L. S., Tangpricha, V., Tishelma, A. c., Van Trotsenburg, M. A. A., Winter, S., Ducheny, K., Adams, N. J., Adrián, T. M., Allen, L. R., Azul, D., Bagga, H., Başar, K., Bathory, D. S., Belinky, J. J., Berg, D. R., Berli, U., Bluebond-Langner, R. O., Bouman, M. B., Bowers, M. L., Brassard, P. L., Byrne, J., Capitán, L., Cargill, C. J., Carswell, J. M., Chang, S. C., Chelvakumar, G., Corneil, T., Dalke, K. B., De Cuypere, G., de Vries, E., Den Heijer, M., Devor, A. H., Dhejne, C., D’Marco, A., Edmiston, E. K., Edwards-Leeper, L., Ehrbar, R., Ehrensaft, D., Eisfeld, J., Elaut, E., Erickson-Schroth, L., Feldman, J. L., Fisher, A. D., Garcia, M. M., Gijs, L., Green, S. E., Hall, B. P., Hardy, T. L. D., Irwig, M. S., Jacobs, L. A., Janssen, A. C., Johnson, K., Klink, D. T., Kreukels, B. P. C., Kuper, L. E., Kvach, E. J., Malouf, M. A., Massey, R., Mazur, T., McLachlan, C., Morrison, S. D., Mosser, S. W., Neira, P. M., Nygren, U., Oates, J. M., Obedin-Maliver, J., Pagkalos, G., Patton, J., Phanuphak, N., Rachlin, K., Reed, T., Rider, G. N., Ristori, J., Robbins-Cherry, S., Roberts, S. A., Rodriguez-Wallberg, K. A., Rosenthal, S. M., Sabir, K., Safer, J. D., Scheim, A. I., Seal, L. J., Sehoole, T. J., Spencer, K., St. Amand, C., Steensma, T. D., Strang, J. F., Taylor, G. B., Tilleman, K., T’Sjoen, G. G., Vala, L. N., Van Mello, N. M., Veale, J. F., Vencill, J. A., Vincent, B., Wesp, L. M., West, M. A., & Arcelus, J. (2022). Standards of care for the health of transgender and gender diverse people, version 8. International Journal of Transgender Health, 23, S1–S259. doi:10.1080/26895269.2022. 2100644
12. Salas-Humara, C., Sequeira, G. M., Rossi, W., & Dhar, C. P. (2019). Gender affirming medical care of transgender youth. Current Problems in Pediatric and Adolescent Health Care, 49(9). doi:10.1016/j.cppeds.2019.100683
13. White Hughto, J. M., & Reisner, S. L. (2016). A systematic review of the effects of hormone therapy on psychological functioning and quality of life in transgender individuals. Transgender Health, 1(1), 21–31. doi:10.1089/trgh.2015.0008
14. Doyle, D. M., Lewis, T. O. G., & Barreto, M. (2023). A systematic review of psychosocial functioning changes after gender-affirming hormone therapy among transgender people. Nature Human Behaviour, 7, 1320–1331. doi:10.1038/s41562-02301605-w
15. Tordoff, D. M., Wanta, J. W., Collin, A., Stepney, C., & Inwards-Breland, D. J. (2022). Mental health outcomes in transgender and nonbinary youths receiving gender-affirming care. JAMA Network Open, 5(2). doi:10.1001/jamanetworkopen.2022.0978
16. Billard, T. J. (2024). The politics of transgender health misinformation. Political Communication, 41(2), 344–352. doi:10.1080/10584609.2024.23031 48
17. Murano-Kinney, S. (2024). Banning puberty-pausing medications endangers transgender adolescents. The American Journal of Bioethics, 24(8), 4–8. doi:10.1080/15265161.2024.2371117
18. Barbee, H., Deal, C., & Gonzales, G. (2022). Anti-transgender legislation—A public health concern for transgender youth. JAMA Pediatrics, 176(2), 125–126. doi:10.1001/jamapediatrics.2021.4483
19. Nwankudu, C. I. (2020). The role of hormones in animal reproduction. Nigerian Veterinary Journal, 41(2), 151–159. doi:10.4314/nvj.v41i2.6
20. Bondesson, M., Hao, R., Lin, C. Y., Williams, C., & Gustafsson, J.-Å. (2015). Estrogen receptor signaling during vertebrate development. Biochimica et Biophysica Acta (BBA) - Gene Regulatory Mechanisms, 1849(2), 160–170. doi:10.1016/j. bbagrm.2014.06.005
21. Yazawa, T., Imamichi, Y., Sato, T., Ida, T., Umezawa, A., & Kitano, T. (2024). Diversity of androgens; Comparison of their significance and characteristics in vertebrate species. Zoological Science, 41(1), 77–86. doi:10.2108/zs230064
22. Di Donato, M., De Falco, P., Cossu, A. M., Abbondanza, C., & Bilancio, A. (2023). Estrogen signaling and breast cancer: GPER, ERa, and microRNAs. Cancers, 15(1), 245. doi:10.3390/cancers15010245
23. Draper, C. F., Duisters, K., Weger, B. D., Chakrabarti, A., Harms, A. C., Brennan, L., Hankemeier, T., van der Greef, J., & van Ommen, B. (2018). Menstrual cycle rhythmicity: Metabolic patterns in healthy women. Scientific Reports, 8(1). doi:10.1038/s41598-018-32647-0
24. Rey, R. A., & Grinspon, R. P. (2020). Androgen treatment in adolescent males with hypogonadism. American Journal of Men’s Health, 14(3). doi:10.1177/1557988320922443
25. Davey, R. A., & Grossmann, M. (2016). Androgen receptor structure, function and biology: From bench to bedside. Clinical Biochemist Reviews, 37(1), 3–15. doi:10.33176/cbr.v37.1.2016.3
26. Almeida, M., Laurent, M. R., Dubois, V., Claessens, F., O’Brien, C. A., Bouillon, R., Vanderschueren, D., & Manolagas, S. C. (2017). Estrogens and androgens in skeletal physiology and pathophysiology. Physiological Reviews, 97(1), 135–187. doi:10.1152/ physrev.00033.2015
27. Mauvais-Jarvis, F., & Lindsey, S. H. (2024). Metabolic benefits afforded by estradiol and testosterone in both sexes: Clinical considerations. Journal of Clinical Investigation, 134(17), e180073. doi:10.1172/JCI180073
28. Aubead, N. M. (2021). Role of sex hormones in human body. In: Courtney Marsh (eds.) Reproductive Hormones, 1–16. IntechOpen. doi:10.5772/ intechopen.95778
29. Sebo, Z. L., & Rodeheffer, M. S. (2021). Testosterone metabolites differentially regulate obesogenesis and fat distribution. Molecular Metabolism, 44. doi:10.1016/j.molmet.2020.101141
30. Alexander, S. E., Gatto, B., Knowles, O. E., Williams, R. M., Fiebig, K. N., Jansons, P., Della Gatta, P., Garnham, A., Eynon, N., Wadley, G. D., Aisbett, B., Hiam, D., & Lamon, S. (2024). Bioavailable testosterone and androgen receptor activation, but not total testosterone, are associated with muscle mass and strength in females. The Journal of Physiology, 602(20), 4567–4589. doi:10.1113/ JP286803
31. Bendis, P. C., Zimmerman, S., Onisiforou, A., Zanos, P., & Georgiou, P. (2024). The impact of estradiol on serotonin, glutamate, and dopamine systems. Frontiers in Neuroscience, 18. doi:10.3389/fnins.2024.1348551
32. Costa, R., & Colizzi, M. (2016). The effect of cross-sex hormonal treatment on gender dysphoria individuals’ mental health: A systematic review. Neuropsychiatric Disease and Treatment, 12, 1953–1966. doi:10.2147/NDT.S95310
33. Roney, J. R., & Simmons, Z. L. (2015). Elevated psychological stress predicts reduced estradiol concentrations in young women. Adaptive Human Behavior and Physiology, 1(1), 30–40. doi:10.1007/s40750-014-0004-2
34. Hwang, W. J., Lee, T. Y., Kim, N. S., & Kwon, J. S. (2020). The role of estrogen receptors and their signaling across psychiatric disorders. International Journal of Molecular Sciences, 22(1). doi:10.3390/ijms22010373
35. Sbisa, A., van den Buuse, M., & Gogos, A. (2018). The effect of estrogenic compounds on psychosis-like behaviour in female rats. PLOS One, 13(3), e0193853. doi:10.1371/journal.pone.0193853
36. Arevalo, M. A., Azcoitia, I., Gonzalez-Burgos, I., & Garcia-Segura, L. M. (2015). Signaling mechanisms mediating the regulation of synaptic plasticity and memory by estradiol. Hormones and Behavior, 74, 19–27. doi:10.1016/j.yhbeh.2015.04.016
37. Krolick, K. N., Zhu, Q., & Shi, H. (2018). Effects of estrogens on central nervous system neurotransmission: Implications for sex differences in mental disorders. Progress in Molecular Biology and Translational Science, 160, 105–171. doi:10.1016/bs.pmbts.2018.07.008
38. Grannis, C., Mattson, W. I., Leibowitz, S. F., Nahata, L., Chen, D., Strang, J. F., Thobe, H., Indyk, J. A., & Nelson, E. E. (2023). Expanding upon the relationship between gender-affirming hormone therapy, neural connectivity, mental health, and body image dissatisfaction. Psychoneuroendocrinology, 156. doi:10.1016/j.psyneuen.2023.106319
39. Achille, C., Taggart, T., Eaton, N. R., Osipoff, J., Tafuri, K., Lane, A., & Wilson, T. A. (2020). Longitudinal impact of gender-affirming endocrine intervention on the mental health and well-being of transgender youths: Preliminary results. International Journal of Pediatric Endocrinology, 2020(1). doi:10.1186/s13633-020-00078-2
40. Barth, C., Villringer, A., & Sacher, J. (2015). Sex hormones affect neurotransmitters and shape the adult female brain during hormonal transition periods. Frontiers in Neuroscience, 9, 37. doi:10.3389/fnins.2015.00037
41. Eliot, L., Ahmed, A., Khan, H., & Patel, J. (2021). Dump the “dimorphism”: Comprehensive synthesis of human brain studies reveals few male-female differences beyond size. Neuroscience & Biobehavioral Reviews, 125, 667–697. doi:10.1016/j. neubiorev.2021.02.026
42. Yoest, K. E., Cummings, J. A., & Becker, J. B. (2014). Estradiol, dopamine and motivation. Central Nervous System Agents in Medicinal Chemistry, 14(2), 83–89. doi:10.2174/187152491466614122 6103135
43. Kranz, G. S., Zhang, B. B. B., Handschuh, P., Ritter, V., & Lanzenberger, R. (2020). Gender-affirming hormone treatment – A unique approach to study the effects of sex hormones on brain structure and function. Cortex, 129, 68–79. doi:10.1016/j.cortex.2020.04.005
44. Eliot, L. (2024). Remembering the null hypothesis when searching for brain sex differences. Biology of Sex Differences, 15. doi:10.1186/s13293-02400585-4
45. Zell, E., Krizan, Z., & Teeter, S. R. (2015). Evaluating gender similarities and differences using metasynthesis. American Psychologist, 70(1), 10–20. doi:10.1037/a0038208
46. Joel, D., & Fausto-Sterling, A. (2016). Beyond sex differences: new approaches for thinking about variation in brain structure and function. Philosophical Transactions of the Royal Society B: Biological Sciences, 371(1688). doi:10.1098/ rstb.2015.0451
47. Joel, D., Persico, A., Salhov, M., Berman, Z., Oligschläger, S., Meilijson, I., & Averbuch, A. (2018). Analysis of human brain structure reveals that the brain “types” typical of males are also typical of females, and vice versa. Frontiers in Human Neuroscience, 12. doi:10.3389/fnhum.2018.00399
48. Joel, D., Berman, Z., Tavor, I., Wexler, N., Gaber, O., Stein, Y., Shefi, N., Pool, J., Urchs, S., Margulies, D. S., Liem, F., Hänggi, J., Jäncke, L., & Assaf, Y. (2015). Sex beyond the genitalia: The human brain mosaic. Proceedings of the National Academy of Sciences, 112(50), 15468–15473. doi:10.1073/ pnas.1509654112
49. Handschuh, P. A., Kranz, G. S., Spies, M., & Lanzenberger, R. (2024). Effects of gender-affirming hormone therapy on gray matter density and microstructure: A longitudinal neuroimaging study. NeuroImage, 291. doi:10.1016/j.neuroimage.2024.120430
50. Seiger, R., Hahn, A., Hummer, A., Kranz, G. S., Ganger, S., Spies, M., Kaufmann, U., Winkler, D., Kasper, S., & Lanzenberger, R. (2016). Subcortical gray matter changes in transgender subjects after long-term cross-sex hormone administration. Psychoneuroendocrinology, 74, 371–379. doi:10.1016/j.psyneuen.2016.09.028
51. Hahn, A., Kranz, G. S., Sladky, R., Kaufmann, U., Ganger, S., Hummer, A., Seiger, R., Spies, M., Vanicek, T., Winkler, D., Kasper, S., Windischberger, C., Swaab, D. F., & Lanzenberger, R. (2016). Testosterone affects language areas of the adult human brain. Human Brain Mapping, 37(5), 1738–1748. doi:10.1002/hbm.23133
52. DeCasien, A. R., Guma, E., Liu, S., & Raznahan, A. (2022). Sex differences in the human brain: A roadmap for more careful analysis and interpretation of a biological reality. Biology of Sex Differences, 13, 43. doi:10.1186/s13293-022-00448-w
53. Ritchie, S. J., Cox, S. R., Shen, X., Lombardo, M. V., Reus, L. M., Alloza, C., Harris, M. A., Alderson, H. L., Hunter, S., Neilson, E., Liewald, D. C. M., Auyeung, B., Whalley, H. C., Lawrie, S. M., Gale, C. R., Bastin, M. E., McIntosh, A. M., & Deary, I. J. (2018). Sex differences in the adult human brain: Evidence from 5216 UK Biobank participants. Cerebral Cortex, 28(8), 2959–2975. doi:10.1093/ cercor/bhy109
54. Marwha, D., Halari, M., & Eliot, L. (2017). Meta-analysis reveals a lack of sexual dimorphism in human amygdala volume. NeuroImage, 147, 282–294. doi:10.1016/j.neuroimage.2016.12.016
55. Tan, A., Ma, W., Vira, A., Marwha, D., & Eliot, L. (2016). The human hippocampus is not sexually-dimorphic: Meta-analysis of structural MRI volumes. NeuroImage, 124, 350–366. doi:10.1016/j. neuroimage.2015.07.061
56. Nave, G., Jung, W. H., Karlsson Linnér, R., Kable, J. W., & Koellinger, P. D. (2018). Are bigger brains smarter? Evidence from a large-scale preregistered study. Psychological Science, 29(8), 1352–1364. doi:10.1177/0956797618808470
57. Lee, J. J., McGue, M., Iacono, W. G., Michael, A. M., & Chabris, C. F. (2019). The causal influence of brain size on human intelligence: Evidence from within-family phenotypic associations and GWAS modeling. Intelligence, 75, 48–58. doi:10.1016/j. intell.2019.01.011
58. Nguyen, H. B., Loughead, J., Lipner, E., Hantsoo, L., Kornfield, S. L., & Epperson, C. N. (2019). What has sex got to do with it? The role of hormones in the transgender brain. Neuropsychopharmacology, 44(1), 22–37. doi:10.1038/s41386-0180140-7
59. Thurston, L. T., Skorska, M. N., Lobaugh, N. J., Zucker, K. J., Chakravarty, M. M., Lai, M.-C., Chavez, S., & VanderLaan, D. P. (2024). White matter microstructure in transmasculine and cisgender adolescents: A multiparametric and multivariate study. PLOS One, 19(3). doi:10.1371/ journal.pone.0300139
60. Guillamon, A., Junque, C., & Gómez-Gil, E. (2016). A review of the status of brain structure research in transsexualism. Archives of Sexual Behavior, 45(7), 1615–1648. doi:10.1007/s10508-016-0768-5
61. Collet, S., Bhaduri, S., Kiyar, M., T’Sjoen, G., Mueller, S., & Guillamon, A. (2021). Characterization of the 1H-MRS metabolite spectra in transgender men with gender dysphoria and cisgender people. Journal of Clinical Medicine, 10(12), 2623. doi:10.3390/jcm10122623
62. Joel, D., Berman, Z., Tavor, I., Wexler, N., Gaber, O., Stein, Y., Shefi, N., Pool, J., Urchs, S., Margulies, D. S., Liem, F., & Hänggi, J. (2015). Sex beyond the genitalia: The human brain mosaic. Proceedings of the National Academy of Sciences, 112(50), 15468–15473. doi:10.1073/pnas.1509654112
63. Sudhakar, D., Huang, Z., Zietkowski, M., Powell, N., & Fisher, A. R. (2023). Feminizing gender-affirming hormone therapy for the transgender and gender diverse population: An overview of treatment modality, monitoring, and risks. Neurourology and Urodynamics, 42(5), 903–920. doi:10.1002/nau.25097
64. Sehgal, I. (2023). Review of adult gender transition medications: Mechanisms, efficacy measures, and pharmacogenomic considerations. Frontiers in Endocrinology, 14. doi:10.3389/fendo.2023.1184024
65. Glintborg, D., T’Sjoen, G., Ravn, P., & Andersen, M. S. (2021). Optimal feminizing hormone treatment in transgender people. European Journal of Endocrinology, 185(2), R49–R63. doi:10.1530/EJE21-0059
66. Tangpricha, V., & den Heijer, M. (2017). Oestrogen and anti-androgen therapy for transgender women. The Lancet Diabetes & Endocrinology, 5(4), 291–300. doi:10.1016/S2213-8587(16)30319-9
67. Yamashina, M., Tsutsui, T., Sei, Y., Akita, M., & Yoshizawa, M. (2019). A polyaromatic receptor with high androgen affinity. Science Advances, 5(4). doi:10.1126/sciadv.aav3179
68. Cheung, A. S., Zwickl, S., Miller, K., Nolan, B. J., Wong, A. F. Q., Jones, P., & Eynon, N. (2024). The impact of gender-affirming hormone therapy on physical performance. The Journal of Clinical Endocrinology & Metabolism, 109(2), e455–e465. doi:10.1210/clinem/dgad414
69. Dimakopoulou, A., & Seal, L. J. (2024). Testosterone and other treatments for transgender males and non-binary individuals: A comprehensive review. Best Practice & Research Clinical Endocrinology & Metabolism, 38(5). doi:10.1016/j. beem.2024.101908
70. Unger, C. A. (2016). Hormone therapy for transgender patients. Translational Andrology and Urology, 5(6), 877–884. doi:10.21037/ tau.2016.09.04
71. Chan, K. J., Jolly, D., Liang, J. J., Weinand, J. D., & Safer, J. D. (2018). Estrogen levels do not rise with testosterone treatment for transgender men. Endocrine Practice, 24(4), 329–333. doi:10.4158/EP-2017-0203
72. Betsi, G., Goulia, P., Sandhu, S., & Xekouki, P. (2024). Puberty suppression in adolescents with gender dysphoria: An emerging issue with multiple implications. Frontiers in Endocrinology, 15. doi:10.3389/fendo.2024.1309904
73. Guss, C., & Gordon, C. M. (2022). Pubertal blockade and subsequent gender-affirming therapy. JAMA Network Open, 5(11). doi:10.1001/jamanetworkopen.2022.39763
74. Olson-Kennedy, J., Streeter, L. H., Garofalo, R., Chan, Y. M., & Rosenthal, S. M. (2021). Histrelin implants for suppression of puberty in youth with gender dysphoria: A comparison of 50 mcg/ day (Vantas) and 65 mcg/day (SupprelinLA). Transgender Health, 6(1), 36–42. doi:10.1089/ trgh.2020.0055
75. Rafferty, J. (2018). Ensuring comprehensive care and support for transgender and gender-diverse Children and adolescents. Pediatrics, 142(4). doi:10.1542/peds.2018-2162
76. Nos, A. L., Klein, D. A., Adirim, T. A., Schvey, N. A., Hisle-Gorman, E., Susi, A., & Roberts, C. M. (2022). Association of gonadotropin-releasing hormone analogue use with subsequent use of gender-affirming hormones among transgender adolescents. JAMA Network Open, 5(11). doi:10.1001/jamanetworkopen.2022.39758
77. Wittlin, N. M., Gallagher, N. M., Atwood, S., & Olson, K. R. (2025). Mental health during medical transition in a US and Canadian sample of early socially transitioned transgender youth. Journal of Adolescent Health, 76(2), 228–237. doi:10.1016/j.jadohealth.2024.10.023
78. van der Miesen, A. I. R., Steensma, T. D., de Vries, A. L. C., Bos, H., & Popma, A. (2020). Psychological functioning in transgender adolescents before and after gender-affirmative care compared with cisgender general population peers. Journal of Adolescent Health, 66(6), 699–704. doi:10.1016/j.jadohealth.2019.12.018
79. Baxendale, S. (2024). The impact of suppressing puberty on neuropsychological function. Authorea, 113(6):1156-1167. doi:10.1111/apa.17150
80. Kilpatrick, L. A., Holmberg, M., Manzouri, A., & Savic, I. (2019). Cross sex hormone treatment is linked with a reversal of cerebral patterns associated with gender dysphoria to the baseline of cisgender controls. European Journal of Neuroscience, 50(8), 3269–3281. doi:10.1111/ejn.14420
81. Holmes, D. (2016). Cross-sex hormones alter grey matter structures. Nature Reviews Endocrinology, 12. doi:10.1038/nrendo.2016.177
82. Burke, S. M., Manzouri, A. H., Dhejne, C., Bergström, K., Arver, S., Feusner, J. D., & Savic-Berglund, I. (2018). Testosterone effects on the brain in transgender men. Cerebral Cortex, 28(5), 1582–1596. doi:10.1093/cercor/bhx054
83. van Eijk, L., Hansell, N. K., Strike, L. T., Couvy-Duchesne, B., de Zubicaray, G. I., Thompson, P. M., & Wright, M. J. (2020). Region-specific sex differences in the hippocampus. NeuroImage, 215. doi:10.1016/j.neuroimage.2020.116781
84. Spindler, M., & Thiel, C. M. (2022). Hypothalamic microstructure and function are related to body mass, but not mental or cognitive abilities across the adult lifespan. GeroScience, 45(1), 277–291. doi:10.1007/s11357-022-00630-3
85. Karalexi, M. A., Georgiopoulos, A. M., & Papageorgiou, C. C. (2020). Gender-affirming hormone treatment and cognitive function in transgender individuals: A systematic review. Psychoneuroendocrinology, 119. doi:10.1016/j.psyneuen.2020.104754
86. Puckett, J. A., Dyar, C., Maroney, M. R., Mustanski, B., & Newcomb, M. E. (2023). Daily experiences of minority stress and mental health in transgender and gender-diverse individuals. Journal of Psychopathology and Clinical Science, 132(3), 340–350. doi:10.1037/abn0000814
87. Mason, A., Crowe, E., Haragan, B., Smith, S., & Kyriakou, A. (2023). Gender dysphoria in young people: A model of chronic stress. Hormone Research in Paediatrics, 96(1), 54–65. doi:10.1159/000520361
88. DuBois, L. Z., Puckett, J. A., Jolly, D., Powers, S. I., Walker, T., Hope, D. A., Mocarski, R., Huit, T. Z., Lash, B. R., Holt, N. R., Ralston, A., Miles, M., Capannola, A., Tipton, C., Eick, G., & Juster, R.-P. (2024). Gender minority stress and diurnal cortisol profiles among transgender and gender diverse people in the United States. Hormones and Behavior, 159, 105473. doi:10.1016/j. yhbeh.2023.105473
89. Turban, J. L., King, D., Kobe, J., Reisner, S. L., & Keuroghlian, A. S. (2022). Access to gender-affirming hormones during adolescence and mental health outcomes among transgender adults. PLOS One, 17(1). doi:10.1371/journal.pone.0261039
90. Correro, A. N., II, & Nielson, K. A. (2020). A review of minority stress as a risk factor for cognitive decline in lesbian, gay, bisexual, and transgender (LGBT) elders. Journal of Gay & Lesbian Mental Health, 24(1), 2–19. doi:10.1080/19359705.2019.164 4570
91. Kidd, J. D., Tettamanti, N. A., Kaczmarkiewicz, R., Corbeil, T. E., Dworkin, J. D., Jackman, K. B., Hughes, T. L., Bockting, W. O., & Meyer, I. H. (2023). Prevalence of substance use and mental health problems among transgender and cisgender U.S. adults: Results from a national probability sample. Psychiatry Research, 326. doi:10.1016/j.psychres.2023.115339
92. Bränström, R., Stormbom, I., Bergendal, M., & Pachankis, J. E. (2022). Transgender-based disparities in suicidality: A population-based study of key predictions from four theoretical models. Suicide and Life-Threatening Behavior, 52(3), 401–412. doi:10.1111/sltb.12830
93. Wolford-Clevenger, C., Cannon, C. J., Flores, L. Y., Smith, P. N., & Stuart, G. L. (2017). Suicide risk among transgender people: A prevalent problem in critical need of empirical and theoretical research. Violence and Gender, 4(3), 69–72. doi:10.1089/vio.2017.0006
94. Green, A. E., DeChants, J. P., Price, M. N., & Davis, C. K. (2022). Association of gender-affirming hormone therapy with depression, thoughts of suicide, and attempted suicide among transgender and nonbinary youth. Journal of Adolescent Health, 70(4), 643–649. doi:10.1016/j. jadohealth.2021.10.036
95. Khorashad, B. S., Manzouri, A., Feusner, J. D., & Savic, I. (2021). Cross-sex hormone treatment and own-body perception: Behavioral and brain connectivity profiles. Scientific Reports, 11. doi:10.1038/s41598-020-80687-2
96. Moody, T. D., Feusner, J. D., Reggente, N., Vanhoecke, J., Holmberg, M., Manzouri, A., Khorashad, B. S., & Savic, I. (2021). Predicting outcomes of cross-sex hormone therapy in transgender individuals with gender incongruence based on pre-therapy resting-state brain connectivity. NeuroImage: Clinical, 29. doi:10.1016/j. nicl.2020.102517
1. Rizvi, S. A. A., Saleh, A. M., Frimpong, H., Al Mohiy, H. M., Ahmed, J., Edwards, R. D., & Ahmed, S. S. (2016). Neurocysticercosis: A case report and brief review. Asian Pacific Journal of Tropical Medicine, 9(1), 100–102. doi:10.1016/j.apjtm.2015.12.020
2. Gripper, L. B., & Welburn, S. C. (2017). Neurocysticercosis infection and disease–A Review. Acta Tropica, 166, 218–224. doi:10.1016/j.actatropica.2016.11.015
3. Hossain, M. S., Shabir, S., & Toye, P. (2023). Insights into the diagnosis, vaccines, and control of Taenia solium, a zoonotic, neglected parasite. Parasites & Vectors 16. doi:10.1186/s13071-023-05989-6
4. Garcia, H. H, Gonzalez, A. E, & Gilman, R. H. (2020). Taenia solium Cysticercosis and Its Impact in Neurological Disease. Clinical Microbiology Reviews, 33. doi:10.1128/cmr.00085-19
5. Demis, C., Anteneh, M., & Basith, A. (2015). Tapeworms of poultry in Ethiopia: A review. British Journal of Poultry Sciences, 4(3), 44-52. doi:10.5829/ idosi.bjps.2015.4.3.96145
6. Butala, C., Brook, T. M., Majekodunmi, A. O., & Welburn, S. C. (2021). Neurocysticercosis: Current perspectives on diagnosis and management. Frontiers in Veterinary Science, 8. doi:10.3389/ fvets.2021.615703
7. Li, L., He, W., Fan, X., Liu, M., Luo, B., Yang, F., Jiang, N., Wang, L., & Zhou, B. (2023). Proteomic analysis of Taenia solium cysticercus and adult stages. Frontiers in Veterinary Science, 9(9). doi:10.3389/ fvets.2022.934197
8. Trevisan, C., Mkupasi, E. M., Ngowi, H. A., Forkman, B. J., & Maria V. (2016). Severe seizures in pigs naturally infected with Taenia solium in Tanzania. Veterinary Parasitology, 20, 67-71. doi:10.1016/j. vetpar.2016.02.025.
9. Carmen-Orozco, Rogger P., Bernal-Teran, Edson G., Chile, Nancy, Condori, Beth J., Cooper, Virginia, Fishbeck, Kayla, Gilman, R. H., Palma, Sandra, Rapport, Laura, Trompeter, Grace, & Verastegui, Manuela. R. (2019). In vitro model of postoncosphere development, and in vivo infection abilities of Taenia solium and Taenia saginata. PLOS Neglected Tropical Diseases, 13(3). doi:10.1371/ journal.pntd.0007261
10. Bustos, J., Gonzales, I., Saavedra, H., Handali, S., & Garcia, H. H. (2021). Neurocysticercosis. A frequent cause of seizures, epilepsy, and other neurological morbidity in most of the world. Journal of the Neurological Sciences, 427, doi:10.1016/J. JNS.2021.117527
11. Marshall, J. S., Warrington, R., Watson, W., & Kim, H. (2018). An introduction to immunology and immunopathology. Allergy Asthma Clin Immunol, 14(S2). doi:10.1186/s13223-018-0278-1
12. Gandhi, N. A., Bennett, B. L., Graham, N. M. H., Pirozzi, G., Stahl, N., & Yancopoulos, G. D. (2016). Targeting key proximal drivers of type 2 inflammation in disease. Nature Reviews Drug Discovery, 15, 35-50. doi:10.1038/nrd4624
13. Prodjinotho, U. F., Lema, J., Lacorcia, M., Schmidt, V., Vejzagic, M., Sikasunge, C., Ngowi, B., Winkler, A. S., & Prazeres da Costa, C. (2020). Host immune responses during Taenia solium neurocysticercosis infection and treatment. PLOS Neglected Tropical Diseases, 14(4), doi:10.1371/journal. pntd.0008005
14. Garcia, H. H., Rodriguez, S., Friedland, J. S. (2014). Immunology of Taenia solium taeniasis and human cysticercosis. Parasite Immunology 36(8), 388-396. doi: 10.1111/pim.12126
15. White, A.C., Jr., Coyle, C. M., Rajshekhar, V., Singh, G., Hauser, W. A., Mohanty, A., Garcia, H. H., & Nash, T. E. (2018). Diagnosis and treatment of neurocysticercosis: 2017 clinical practice guidelines by the Infectious Diseases Society of America (IDSA) and the American Society of Tropical Medicine and Hygiene (ASTMH). Clinical Infectious Diseases, 66(8), 49-75. doi:10.1093/cid/cix1084
16. Abanto, J., Blanco, D., Saavedra, H., Gonzales, I., Siu, D., Pretell, E. J., Bustos, J. A., & Garcia, H. H. (2021). Mortality in parenchymal and subarachnoid neurocysticercosis. The American Journal of Tropical Medicine and Hygiene, 105(1), 176-180. doi:10.4269/ajtmh.20-1330
17. Tadevosyan, A., & Kornbluth, J. (2021). Brain herniation and intracranial hypertension. Neurological Clinics, 39(2), 293-318. doi:10.1016/j. ncl.2021.02.005
18. Ratcliffe, C., Adan, G., Marson, A., Solomon, T., Saini, J., Sinha, S., & Keller, S. S. (2023). Neurocysticercosis-related seizures: Imaging biomarkers. Seizure: European Journal of Epilepsy, 108, 13-23 doi:10.1016/j.seizure.2023.04.005
19. Steyn, T. J. S., Awala, A. N., de Lange, A., & Raimondo, J. V. (2022). What causes seizures in neurocysticercosis? Epilepsy Currents, 23(2), 105-112. doi:10.1177/15357597221137418
20. Schaper, F. L., Nordberg, J., Cohen, A. L., Lin, C., Hsu, J., Horn, A., Ferguson, M. A., Siddiqi, S. H., Drew, W., Soussand, L., Winkler, A. M., Simó, M, Bruna, J., Rheims, S., Guenot, M., Bucci, M., Nummenmaa, L., Staals, J., Colon, A. J., Ackermans, L., Bubrick, E. J., Peters, J. M., Wu, O., Rost, N. S., Grafman, J., Blumenfeld, H., Temel, Y., Rouhl, R. P., Joutsa, J., & Fox, M. D. (2023). Mapping lesion-related epilepsy to a human brain network. JAMA Neurology, 80(9), 891-902. doi:10.1001/jamaneurol.2023.1988
21. Archibald, L. K., & Quisling R. G. (2013). Central nervous system infections. In: Layon, A., Gabrielli, A., & Friedman, W. (eds.) Textbook of Neurointensive Care, 427–517. Springer. doi:10.1007/978-14471-5226-2_22
22. Pal, A., Biswas, C., Ghosh, T., & Deb, P. (2017). A rare case of recurrence of primary spinal neurocysticercosis mimicking an arachnoid cyst. Asian Journal of Neurosurgery, 12(2), 250-252. doi:10.4103/1793-5482.144176
23. Barrie, U., Badejo, O., Aoun, S. G., Adeyemo, E., Moler, N., Christian, Z. K., Caruso, J. P., El Ahmadieh, T. Y., Ban, V. S., MacAllister, M. C., Reyes, V. P., Hall, K., Whitworth, L., & Bagley, C. (2020). Systematic review and meta-analysis of management strategies and outcomes in adult spinal neurocysticercosis. World Neurosurgery, 138, 504-511. doi:10.1016/j.wneu.2020.03.093.
24. Manh, B. H., Dat, T., Hai, V. T., He, D. V., Ha, D. D., Que, N. V., & Duc, N. M. (2023). Spinal cysticercosis: A case report, Radiology Case Reports, 18(9), 3269-3273, doi:10.1016/j.radcr.2023.06.037.
25. Hurła, M., Pikor, D., Kościelecka, K., Drelichowska, A., Banaszek, N., & Paul, M. (2024). Neurocysticercosis—Diagnostic mystery: Current status for Europe. BioMed, 4(3), 302-313; doi:10.3390/ biomed4030024
26. Grover, V. P. B., Tognarelli, J. M., Crossey, M. M. E., Cox, I. J., Taylor-Robinson, S. D., & McPhail, M. J. W. (2015). Magnetic resonance imaging: Principles and techniques: Lessons for clinicians. Journal of Clinical and Experimental Hepatology, 5(3), 246255, doi:10.1016/j.jceh.2015.08.001
27. Tang, N. L., Nash, T. E., Corda, M., Nutman, T. B., & O’Connell E. M. (2023). Triplex ELISA for assessing durability of Taenia solium seropositivity after neurocysticercosis cure. Emerging Infectious Diseases, 29(7),1340-1348. doi:10.3201/ eid2907.230364
28. Arroyo, G., Rodriguez, S., Lescano, A. G., Alroy, K. A., Bustos, J. A, Santivañez, S., Gonzales, I., Saavedra, H., Pretell, E. J., Gonzalez, A. E., Gilman, R. H., Tsang, V. C., & Garcia, H. H. (2018). Antibody banding patterns of the enzyme-linked immunoelectrotransfer blot and brain imaging findings in patients with neurocysticercosis. Clinical Infectious Diseases, 66(2), 282–288. doi:10.1093/cid/cix774
29. Passaro, A., Jänne, P.A., & Peters, S. (2023). Antibody-drug conjugates in lung cancer: recent advances and implementing strategies. Journal of Clinical Oncology, 41(21). doi:10.1200/JCO.23.00013
30. Del Brutto, O. H. (2020). Current approaches to cysticidal drug therapy for neurocysticercosis. Expert Review of Anti-Infective Therapy, 18(8), 789–798. doi:10.1080/14787210.2020.1761332
31. Andrade-Mogrovejo, D. A., Gonzales-Gustavson, E., Ho-Palma, A. C., Prada, J. M., Bonnet, G., Pizzitutti, F., Gomez-Puerta, L. A., Arroyo, G., O’Neal, S. E., Garcia, H. H., Guitian, J., & Gonzalez, A. (2022). Development of a dose-response model for porcine cysticercosis. PLOS One, 17(3). doi:10.1371/ journal.pone.0264898
32. Ramamoorthy, S., & Cidlowski, J.A. (2016). Corticosteroids: Mechanisms of action in health and disease. Rheumatic Disease Clinics of North America, 42(1), 15-31. doi:10.1016/j.rdc.2015.08.002
33. Hamamoto Filho, P. T., Norcia, L. F., Fleury, A., & Zanini, M. A. (2024). Current role of surgery in the treatment of neurocysticercosis. Pathogens, 13(3). doi:10.3390/pathogens13030218
34. Takayanagui, O.M., & Marques de Haes, T. (2022). Update on the diagnosis and management of neurocysticercosis. Arquivos de Neuro-Psiquiatria 80(5). doi:10.1590/0004-282X-ANP-2022-S115
35. Carpio, A., Fleury, A., Romo, M. L., & Abraham, R. (2018). Neurocysticercosis: The good, the bad, and the missing. Expert Review of Neurotherapeutics, 18(4), 289–301. doi:10.1080/14737175.2018.1451328
36. Rajshekhar, V. (2016). Neurocysticercosis. Diagnostic problems & current therapeutic strategies. Indian Journal of Medical Research, 144(3), 319–326. doi:10.4103/0971-5916.198686
37. Sharma, S., Zapatero-Rodríguez, J., Estrela, P., & O’Kennedy, R. (2015). Point-of-care diagnostics in low resource settings: Present status and future role of microfluidics. Biosensors, 5(3), 577-601. doi:10.3390/bios5030577
38. Guzman, C., & Garcia, H. H. (2021). Current diagnostic criteria for neurocysticercosis. Research and Reports in Tropical Medicine, 12, 197–203. doi:10.2147/rrtm.s285393
39. Pollard, A. J., & Bijker, E. M. A. (2021) Guide to vaccinology: From basic principles to new developments. Nature Reviews Immunology, 21, 83–100. doi:10.1038/s41577-020-00479-7
40. Stutzer, C., Richards, S. A., Ferriera, M., Baron, S., & Maritz-Olivier, C. (2018). Metazoan parasite vaccines: Present status and future prospects. Frontiers in Cellular and Infection Microbiology, 8. doi:10.3389/fcimb.2018.00067
41. Carpio, A., Romo, M. L., Parkhouse, R. M. E., Short, B., & Dua, T. (2016). Parasitic diseases of the central nervous system: Lessons for clinicians and policy makers. Expert Review of Neurotherapeutics, 16(4), 404-414. doi:10.1586/14737175.2016.115 5454
FROM HEALING
DEITIES TO HEALTHCARE DIFFICULTIES: THE STRUGGLE TO UNDERSTAND FUNCTIONAL SOMATIC DISORDERS
1. Abdelnaim, M. A., Hebel, T., Lang-Hambauer, V., Schlaier, J., Langguth, B., & Reissmann, A. (2025). Deep brain stimulation for obsessive compulsive disorder leads to symptom changes of comorbid irritable bowel syndrome. Frontiers in Psychiatry, 16. doi:10.3389/fpsyt.2025.1545318
2. Aman, M. M., Yong, R. J., Kaye, A. D., Urman, R. D. (2018). Evidence-based non-pharmacological therapies for fibromyalgia. Current Pain and Headache Reports, 22(33). doi:10.1007/s11916018-0688-2
3. Antunes, M. D., & Marques, A. P. (2022). The role of physiotherapy in fibromyalgia: Current and future perspectives. Front. Psychiatry, 13. doi:10.3389/fphys.2022.968292
4. Baizabal-Carvallo, J. F., Hallett, M., & Jankovic, J. (2019). Pathogenesis and pathophysiology of functional (psychogenic) movement disorders. Neurobiology of Disease, 127, 32-44. doi:10.1016/j. nbd.2019.02.013
5. Berezowski, L., Ludwig, L., Martin, A., Lowe, B., & Shedden-Mora, M. C. (2022). Early psychological interventions for somatic symptom disorder and functional somatic syndromes: A systematic review and meta-analysis. Psychosomatic Medicine, 84(3), 325-338. doi:10.1097/ PSY.0000000000001011
6. Black, C. J., Drossman, D. A., Talley, N. J., Ruddy, J., & Ford, A. C. (2020). Functional gastrointestinal disorders: advances in understanding and management. The Lancet, 396(10263), 1664-1674. doi:10.1016/S0140-6736(20)32115-2
7. Blakemore, R. L., Sinanaj, I., Galli, S., Aybek, S., & Vuilleumier, P. (2016). Aversive stimuli exacerbate defensive motor behaviour in motor conversion disorder. Neuropsychologia, 93, 229-241. doi:10.1016/j.neuropsychologia.2016.11.005
8. Boylan, K. A., Dworetzky, B. A., Baslet, G., Polich, G., O’Neal, M. A., & Reinsberger, C. (2024). Functional neurological disorder, physical activity and exercise: What we know and what we can learn from comorbid disorders. Epilepsy & Behavior Reports, 27. doi:10.1016/j.ebr.2024.100682
9. Budtz-Lilly, A., Schroder, A., Rask, M. T., Fink, P., Vestergaard, M., & Rosendal, M. (2015). Bodily distress syndrome: A new diagnosis for functional disorders in primary care? BMC Family Practice, 16(180). doi:10.1186/s12875-015-0393-8
10. Burton, C., Fink, P., Henningsen, P., Lowe, B., & Rief, W. (2020). Functional somatic disorders: Discussion paper for a new common classification for research and clinical use. BMC Medicine, 18(34). doi:10.1186/s12916-020-1505-4
11. Califf, R. M. (2018). Biomarker definitions and their applications. Experimental Biology and Medicine, 243(3). doi:10.1177/1535370217750088
12. Calma, A. D., Heffernan, J., Farrell, N., Gelauff, J., O’Connell, N., Perez, D. L., Perriman, D., Smyth, L., Stone, J., & Lueck, C. J. (2023). The impact of depression, anxiety and personality disorders on the outcome of patients with functional limb weakness – Individual patient data meta-analysis. Journal of Psychosomatic Research, 175. doi:10.1016/j.jpsychores.2023.111513
13. Chen, D. K., Sharma, E., & LaFrance, W. C. (2017). Psychogenic non-epileptic seizures. Current Neurology and Neuroscience Reports, 17(71). doi:10.1007/s11910-017-0781-7
14. Conde-Antón, A., Hernando-Garijo, I., Jiménezdel-Barrio, S., Mingo-Gómez, M. T., Medrano-de-la-Fuente, R., & Ceballos-Laita, L. (2023). Effects of transcranial direct current stimulation and transcranial magnetic stimulation in patients with fibromyalgia. A systematic review. Neurología, 38(6), 427-439. doi:10.1016/j.nrleng.2020.07.025
15. Czachowski, S. (2023). Functional disordersnew proposals for definition, psychosomatics, and somatization. Psychiatria Polska, 57(2), 421430. doi:10.12740/PP/OnlineFirst/141960
16. David, D. J., & Gourion, D. (2016). Antidepressant and tolerance: Determinants and management of major side effects. L’Encephale, 42(6), 553-561. doi:10.1016/j.encep.2016.05.006
17. Driessen, E., Hegelmaier, L. M., Abbass, A. A., Barber, J. P., Dekker, J. J. M., Van, H. L., Jansma, E. P., & Cuijpers, P. (2015). The efficacy of shortterm psychodynamic psychotherapy for depression: A meta-analysis update. Clinical Psychology Review, 42, 1-15. doi:10.1016/j.cpr.2015.07.004
18. Donnachie, E., Schneider, A., & Enck, P. (2020). Comorbidities of patients with functional somatic syndromes before, during and after first diagnosis: A population-based study using bavarian routine data. Scientific Reports, 10. doi:10.1038/ s41598-020-66685-4
19. Dunphy, L., Penna, M., & El-Kafsi, J. (2019). Somatic symptom disorder: A diagnostic dilemma. BMJ Case Reports, 12(11). doi:10.1136/bcr-2019231550
20. Fernandez-Feijoo, F., Samartin-Veiga, N., & Carrillo-de-la-Pena, M. T. (2022). Quality of life in patients with fibromyalgia: Contributions of disease symptoms, lifestyle and multi-medication. Frontiers in Psychology, 13. doi:10.3389/ fpsyg.2022.924405
21. Fu, X., Zhang, F., Liu, F., Yan, C., & Guo, W. (2019). Editorial: Brain and somatization symptoms in psychiatric disorders. Frontiers in Psychiatry, 10. doi:10.3389/fpsyt.2019.00146
22. Gálvez-Sánchez, C. M., Duschek, S., & Reyes del Paso, G. A. (2019). Psychological impact of fibromyalgia: current perspectives. Psychology Research and Behavior Management, 12, 117-127. doi:10.2147/PRBM.S178240
23. Gilmour, G. S., Nielsen, G., Teodoro, T., Yogarajah, M., Coebergh, J. A., Dilley, M. D., Martino, D., & Edwards, M. J. (2020). Management of functional neurological disorder. Journal of Neurology, 267, 2164-2172. doi:10.1007/s00415-020-09772-w
24. Gogolla, N. (2017). The insular cortex. Current Biology, 27(12), 580-586. doi:10.1016/j. cub.2017.05.010
25. Gonsalvez, I., Spagnolo, P., Dworetzky, B., & Baslet, G. (2021). Neurostimulation for the treatment of functional neurological disorder: A systematic review. Epilepsy & Behavior Reports, 16. doi:10.1016/j.ebr.2021.100501
26. Gutkin, M., McLean, L., Brown, R., & Kanaan, R. A. (2021). Systematic review of psychotherapy for adults with functional neurological disorder. Journal of Neurology, Neurosurgery & Psychiatry, 92, 36-44. doi:10.1136/jnnp-2019-321926
27. Hackshaw, K. V. (2021). The search for biomarkers in fibromyalgia. Diagnostics, 11(2). doi:10.3390/diagnostics11020156
28. Harmer, C. J., Duman, R. S., & Cowen, P. J. (2017). How do antidepressants work? New perspectives for refining future treatment approaches. Lancet Psychiatry, 4(5), 409-418. doi:10.1016/S22150366(17)30015-9
29. Hauser, W., Fitzcharles, M., & Henningsen, P. (2025). Fibromyalgia syndrome—A bodily distress disorder/somatic symptom disorder? Pain Reports, 10(1). doi:10.1097/PR9.0000000000001223
30. Henningsen, P. (2022). Management of somatic symptom disorder. Dialogues in Clinical Neuroscience 20(1), 23-31. doi:10.31887/DCNS.2018.20.1/ phenningsen
31. Heseltine-Carp, W., Dale, V., van Eck van der Sluijs, J., & van der Feltz-Cornelis, C. (2023). Are serum hsCRP and IL-6 prognostic markers in somatic symptom disorder and related disorders? An exploratory analysis in a prospective cohort study. Journal of Psychiatric Research, 157, 8895. doi:10.1016/j.jpsychires.2022.11.026
32. Jenkins, W., & Smart, K. (2020). Somatization in acute care pediatrics: Respecting the mind-body connection. Clinical Child Psychology and Psychiatry, 25(3). doi:10.1177/1359104520905065
33. Kano, M., Oudenhove, L. V., Dupont, P., Wager, T. D., & Fukudo, S. (2020). Imaging brain mechanisms of functional somatic syndromes: Potential as a biomarker? The Tohoku Journal of Experimental Medicine, 250(3), 137-152. doi:10.1620/ tjem.250.137
34. Kazantzis, N., Luong, H. K., Usatoff, A. S., Impala, T., Yew, R. Y., & Hofmann, S. G. (2018). The processes of cognitive behavioral therapy: A review of meta-analyses. Cognitive Therapy and Research, 42, 349-357. doi:10.1007/s10608-0189920-y
35. Khalsa, S. S., Adolphs, R., Cameron, O. G., Critchley, H. D., Davenport, P. W., Feinstein, J. S., Feusner, J. D., Garfinkel, S. N., Lane, R. D., Mehling, W. E., Meuret, A. E., Nemeroff, C. B., Oppenheimer, S., Petzschner, F. H., Pollatos, O., Rhudy, J. L., Schramm, L. P., Simmons, W. K., Stein, M. B., Stephan, K. E., & Zucker, N. (2018). Biology Psychiatry: Cognitive Neuroscience and Neuroimaging, 3(6), 501-513. doi:10.1016/j.bpsc.2017.12.004
36. Kim, J. H., Lin, E., & Pimentel, M. (2017). Biomarkers of irritable bowel syndrome. Journal of Neurogastroenterology and Motility, 23(1), 20-26. doi:10.5056/jnm16135
37. Kleykamp, B. A., Ferguson, M. C., McNicol, E., Bixho, I., Arnold, L. M., Edwards, R. R., Fillingim, R., Grol-Prokopczyk, H., Turk, D. C., & Dworkin, R. H. (2021). The prevalence of psychiatric and chronic pain comorbidities in fibromyalgia: An ACTTION systematic review. Seminars in Arthritis and Rheumatism, 51(1), 166-174. doi:10.1016/j.semarthrit.2020.10.006
38. Koh, K. B. (2018). Biological Mechanisms of Somatization. In: Stress and Somatic Symptoms, 95-103. Springer. doi:10.1007/978-3-030-027834_9
39. Kozlowska, K., Scholar-Root, O., Savage, B., Hawkes, C., Chudleigh, C., Raghunandan, J., Scher, S., & Helgeland, H. (2023). Illness-promoting psychological processes in children and adolescents with functional neurological disorder. Children, 10(11). doi:10.3390/children10111724
40. Labrakakis, C. (2023). The role of the insular cortex in pain. International Journal of Molecular Sciences, 24(6). doi:10.3390/ijms24065736
41. Lagrand, T. J., Jones, M., Bernard, A., & Lehn, A. C. (2023). Impact of explaining the diagnosis of functional seizures on healthcare costs. Neurology Clinical Practice, 13(1). doi:10.1212/ CPJ.0000000000200111
42. Lazzari, C., Nikolou-Walker, E., Liu, L. Q., & Rabottini, M. (2024). Psychiatric comorbidities in functional neurological disorders and psychogenic non-epileptic seizures: A systematic review and policy recommendations for improving assessment and treatment. Neuropsychiatric Disease and Treatment, 20, 2313-2331. doi:10.2147/ NDT.S491376
43. Leibetseder, A., Eisermann, M., LaFrance, W. C., Nobili, L., & von Oertzen, T. J. (2021). How to distinguish seizures from non-epileptic manifestations. The Educational Journal of the International League Against Epilepsy, 22(6), 716-738. doi:10.1684/epd.2020.1234
44. León-Pedroza, J. I., González-Tapia, L. A., del Olmo-Gil, E., Castellanos-Rodríguez, D., Escobedo, G., & González-Chávez, A. (2015). Low-grade systemic inflammation and the development of metabolic diseases: From the molecular evidence to the clinical practice. Cirugía y Cirujanos, 83(6), 543-551. doi:10.1016/j.circen.2015.11.008
45. Li, J., Lu, C., Gao, Z., Feng, Y., Luo, H., Lu, T., Sun, X., Hu, J., & Luo, Y. (2020). SNRIs achieve faster antidepressant effects than SSRIs by elevating the concentrations of dopamine in the forebrain. Neuropharmacology, 177. doi:10.1016/j.neuropharm.2020.108237
46. Liao, S., Ma, H., Lin, Y., & Huang, W. (2019). Functioning and quality of life in patients with somatic symptom disorder: The association with comorbid depression. Comprehensive Psychiatry, 90, 88-94. doi:10.1016/j.comppsych.2019.02.004
47. Loge-hagen, J. S., Saele, A., Juhl, C., Bech, P., Stenager, E., & Mellentin, A. I. (2019). Prevalence of depressive disorder among patients with fibromyalgia: Systematic review and meta-analysis. Journal of Affective Disorders, 245, 10981105. doi:10.1016/j.jad.2018.12.001
48. Lowe, B., Levenson, J., Depping, M., Husing, P., Kohlmann, S., Lehmann, M., Shedden-Mora, M., Toussaint, A., Uhlenbusch, N., & Weigel, A. (2021). Somatic symptom disorder: A scoping review on the empirical evidence of a new diagnosis. Psychological Medicine, 52(4), 632-648. doi:10.1017/ S0033291721004177
49. Mark, V. W. (2024). Biomarkers and rehabilitation for functional neurological disorder. Journal of Personalized Medicine, 14(9). doi:10.3390/ jpm14090948
50. Mayor, E. A., Ryu, H. J., & Bhatt, R. R. (2023). The neurobiology of irritable bowel syndrome. Molecular Psychiatry, 28, 1451-1465. doi:10.1038/s41380023-01972-w
51. Mavroudis, I., Kazis, D., Kamal, F. Z., Gurzu, I., Ciobica, A., Padurariu, M., Novac, B., & Iordache, A. (2024). Understanding functional neurological disorder: Recent insights and diagnostic challenges. International Journal of Molecular Sciences, 25(8), 4470. doi:10.3390/ijms25084470
52. McCrae, C. S., Curtis, A. F., Craggs, J., Deroche, C., Sahota, P., Siva, C., Staud, R., & Robinson, M. (2020). Protocol for the impact of CBT for insomnia on pain symptoms and central sensitisation in fibromyalgia: A randomised controlled trial. BMJ Open, 10(9). doi:10.1136/bmjopen-2019-033760
53. Miorelli, A. (2020). Psychiatric aspects of chronic physical disease. Medicine, 48(12), 784-787. doi:10.1016/j.mpmed.2020.09.009
54. Ng, Q. X., Soh, A. Y. S., Loke, W., Lim, D. Y., & Yeo, W. S. (2018). The role of inflammation in irritable bowel syndrome (IBS). Journal of Inflammation Research, 11, 345-349. doi:10.2147/JIR.S174982
55. Olguín, H. J., Guzmán, D. C., García, E. H., & Mejía, G. B. (2015). The role of dopamine and its dysfunction as a consequence of oxidative stress. Oxidative Medicine and Cellular Longevity doi:10.1155/2016/9730467
56. Onofrj, M., Ajdinaj, P., Digiovanni, A., Malek, N., Martinotti, G., Ferro, F. M., Russo, M., Thomas, A., & Sensi, S. L. (2023). Functional neurologic disorders, disorders to be managed by neurologists, or are neurologists wandering in a dangerous field with inadequate resources? Frontiers in Psychiatry, 14. doi:10.3389/fpsyt.2023.1120981
57. Ospina, J. P., Jalilianhasanpour, R., & Perez, D. L. (2019). The role of the anterior and midcingulate cortex in the neurobiology of functional neurologic disorder. Handbook of Clinical Neurology, 166, 267-279. doi:10.1016/B978-0-444-641960.00014-5
58. Perez, D. L., Nicholson, T. R., Asadi-Pooya, A. A., Bègue, I., Butler, M., Carson, A. J., David, A. S., Deeley, Q., Diez, I., Edwards, M. J., Espay, A. J., Gelauff, J. M., Hallett, M., Horovitz, S. G., Jungilligens, J., Kanaan, R. A. A., Tijssen, M. A. J., Kozlowska, K., LaFaver, K., LaFrance, W. C., & Aybek, S. (2021). Neuroimaging in functional neurological disorder: State of the field and research agenda. NeuroImage: Clinical, 30. doi:10.1016/j. nicl.2021.102623
59. Popa, S., & Dumitrascu, D. L. (2015). Anxiety and IBS revisited: Ten years later. Medicine and Pharmacy Reports, 88(3), 253-257. doi:10.15386/ cjmed-495
60. Radziszewska, M., Smarkusz-Zarzecka, J., & Ostrowska, L. (2023). Nutrition, physical activity and supplementation in irritable bowel syndrome. Nutrients, 15(16). doi:10.3390/nu15163662
61. Roelofs, J. J., Teodoro, T., & Edwards, M. J. (2019). Neuroimaging in functional movement disorders. Current Neurology and Neuroscience Reports, 19(12). doi:10.1007/s11910-019-0926-y
62. Rossetti, M. G., Delvecchio, G., Calati, R., Perlini, C., Bellani, M., & Brambilla, P. (2021). Structural neuroimaging of somatoform disorders: A systematic review. Neuroscience & Biobehavioral Reviews, 122, 66-78. doi:10.1016/j.neubiorev.2020.12.017
63. Russell, A., Hepgul, N., Nikkheslat, N., Borsini, A., Zajkowska, Z., Moll, N., Forton, D., Agarwal, K., Chalder, T., Mondelli, V., Hotopf, M., Cleare, A., Murphy, G., Foster, G., Wong, T., Schutze, G. A., Schwarz, M. J., Harrison, N., Zunszain, P. A., & Pariante, C. M. (2019). Persistent fatigue induced by interferon-alpha: A novel, inflammation-based, proxy model of chronic fatigue syndrome. Psychoneuroendocrinology, 100, 276-285. doi:10.1016/j.psyneuen.2018.11.032
64. Schneider, A., Weber, S., Wyss, A., Loukas, S., & Aybek, S. (2024). BOLD signal variability as potential new biomarker of functional neurological disorders. Neuroimage: Clinical, 43. doi:10.1016/j. nicl.2024.103625
65. Schneider, J., Patterson, M., & Jimenez, X. F. (2019). Beyond depression: Other uses for tricyclic antidepressants. Cleveland Clinic Journal of Medicine, 86(12), 807-814. doi:10.3949/ ccjm.86a.19005
66. Schulz, A., & Vögele, C. (2015). Interoception and stress. Frontiers in Psychology, 6. doi:10.3389/ fpsyg.2015.00993
67. Sharie, S. A., Varga, S. J., Al-Husinat, L., Sarzi-Puttini, P., Araydah, M., Bal’awi, B. R., & Varrassi, G. (2024). Unraveling the complex web of fibromyalgia: A narrative review. Medicina, 60(2), 272. doi:10.3390/medicina60020272
68. Shi, X., Hu, Y., Zhang, B., Li, W., Chen, J. D. Z., & Liu, F. (2021). Ameliorating effects and mechanisms of transcutaneous auricular vagal nerve stimulation on abdominal pain and constipation. JCI Insight, 6(14). doi:10.1172/jci.insight.150052
69. Shoeibi, A., Khodatars, M., Ghassemi, N., Jafari, M., Moridian, P., Alizadehsani, R., Panahiazar, M., Khozeimeh, F., Zare, A., Hosseini-Nejad, H., Khosravi, A., Atiya, A. F., Aminshahidi, D., Hussain, S., Rouhani, M., Nahavandi, S., & Acharya, U. R. (2021). Epileptic seizures detection using deep learning techniques: A review. International Journal of Environmental Research and Public Health, 18(11). doi:10.3390/ijerph18115780
70. Stieler, M., Pockney, P., Campbell, C., Thirugnanasundralingam, V., Gan, L., Spittal, M. J., & Carter, G. (2022). Somatic symptom severity association with healthcare utilization and costs in surgical inpatients with an episode of abdominal pain. BJS Open, 6(4). doi:10.1093/bjsopen/ zrac046
71. Staudacher, H. M., Black, C. J., Teasdale, S. B., Mikocka-Walus, A., & Keefer, L. (2023). Irritable bowel syndrome and mental health comorbidity — Approach to multidisciplinary management. Nature Reviews Gastroenterology & Hepatology, 20, 582-596. doi:10.1038/s41575-023-00794-z
72. Todorovic, M., Timotijevic, I., & Crnic, K. (2015). Chronic pain, affective spectrum disorders and treatment with antidepressants. European Psychiatry, 30(1), 1265. doi:10.1016/S09249338(15)30990-1
73. Tornblom, H., & Drossman, D. A. (2021). Psychopharmacologic therapies for irritable bowel syndrome. Gastroenterology Clinics of North America, 50(3), 655-669. doi:10.1016/j.gtc.2021.04.005
74. Treister-Goltzman, Y., & Peleg, R. (2023). Fibromyalgia and mortality: A systematic review and meta-analysis. RMD Open, 9(3). doi:10.1136/rmdopen-2023-003005
75. Ulloa, L., Quiroz-Gonzalez, S., & Torres-Rosas, R. (2017). Nerve stimulation: Immunomodulation and control of inflammation. Trends in Molecular Medicine, 23(12), 1103-1120. doi:10.1016/j. molmed.2017.10.006
76. van der Feltz-Cornelis, C. M., Elfeddali, I., Werneke, U., Malt, U. F., Van den Bergh, O., Schaefert, R., Kop, W. J., Lobo, A., Sharpe, M., Sollner, W., & Löwe, B. (2018). A European research agenda for somatic symptom disorders, bodily distress disorders, and functional disorders: Results of an estimate-talk-estimate Delphi expert study. Frontiers in Psychiatry, 9. doi:10.3389/fpsyt.2018.00151
77. van der Feltz-Cornelis, C. M., Bakker, M., Kaul, A., Kuijpers, T. W., von Kanel, R., & van Eck van der Sluijs, J. F. (2020). IL-6 and hsCRP in somatic symptom disorders and related disorders. Brain, Behavior, & Immunity - Health, 9, doi:10.1016/j. bbih.2020.100176
78. van der Feltz-Cornelis, C., Bakker, M., & van Eck van der Sluijs, J. (2022). Four clinical profiles of adult outpatients with Somatic Symptom Disorders and Related Disorders (SSRD). A latent case analysis. Journal of Psychosomatic Research, 156. doi:10.1016/j.jpsychores.2022.110775
79. Walitt, B., Klose, P., Üçeyler, N., Phillips, T., & Hauser, W. (2016). Antipsychotics for fibromyalgia in adults. Cochrane Database of Systematic Reviews, 6. doi:10.1002/14651858.CD011804.pub2
80. Widyadharma, I. P. E., Soejitno, A., Samatra, D. P. G. P., & Sinardja, A. M. G. (2021). Clinical differentiation of psychogenic non-epileptic seizure: A practical diagnostic approach. The Egyptian Journal of Neurology, Psychiatry and Neurosurgery, 57. doi:10.1186/s41983-021-00272-w
81. Yepez, D., Grandes, X. A., Manjunatha, R. T., Habib, S., & Sangaraju, S. L. (2022). Fibromyalgia and depression: A literature review of their shared aspects. Cureus, 14(5). doi:10.7759/cureus.24909
FEATURED
HARNESSING THE HEAT: NATURE’S FIERY SOLUTION TO PAIN
1. Fattori, V., Hohmann, M. S. N., Rossaneis, A. C., Pinho-Ribeiro, F. A., & Verri, W. A. (2016). Capsaicin: Current understanding of its mechanisms and therapy of pain and other pre-clinical and clinical uses. Molecules, 21(7). doi:10.3390/molecules21070844
2. Basith, S., Cui, M., Hong, S., & Choi, S. (2016). Harnessing the therapeutic potential of capsaicin and its analogues in pain and other diseases. Molecules, 21(8). doi:10.3390/molecules21080966
3. Thomas, S. E., & Laycock, H. (2020). The use of high dose topical capsaicin in the management of peripheral neuropathy: Narrative review and local experience. British Journal of Pain, 14(2), 133-140. doi:10.1177/2049463720914332
4. Finnerup, N. B., Attal, N., Haroutounian, S., McNicol, E., Baron, R., Dworkin, R. H., Gilron, I., Haanpää, M., Hansson, P., Jensen, T. S., Kamerman, P. R., Lund, K., Moore, A., Raja, S. N., Rice, A. S. C., Rowbotham, M., Sena, E., Siddall, P., Smith, B. H., & Wallace, M. (2015). Pharmacotherapy for neuropathic pain in adults: A systematic review and meta-analysis. The Lancet Neurology, 14(2), 162–173. doi:10.1016/s1474-4422(14)70251-0
5. Staelin, R., Koneru, S. N., & Rawe, I. M. (2017) An over-the-counter central sensitization therapy: A chronic back pain registry study of pain relief, medication use and their adverse effects. Pain Management, 7(2), 99-111. doi:10.2217/pmt-20160046
6. Scott, N.L. & Miner, J.R. (2016) The safe and rational use of analgesics: Non-opioid analgesics. Current Emergency and Hospital Medicine Reports, 4, 66–70. doi:10.1007/s40138-016-0102-5
7. Mickle, A. D., Shepherd, A. J., & Mohapatra, D. P. (2016). Nociceptive TRP channels: Sensory detectors and transducers in multiple pain pathologies. Pharmaceuticals, 9(4), 72. doi:10.3390/ ph9040072
8. Fischer, M. J. M., Ciotu, C. I., & Szallasi, A. (2020). The mysteries of capsaicin-sensitive afferents. Frontiers in Physiology, 11. doi:10.3389/ fphys.2020.554195
9. Frias, B., & Merighi, A. (2016). Capsaicin, nociception and pain. Molecules, 21(6). doi:10.3390/molecules21060797
10. Kawakami, S., Sato, H., Sasaki, A. T., Tanabe, H. C., Yoshida, Y., Saito, M., Toyoda, H., Sadato, N., & Kang, Y. (2016). The brain mechanisms underlying the perception of pungent taste of capsaicin and the subsequent autonomic responses. Frontiers in Human Neuroscience, 9. doi:10.3389/ fnhum.2015.00720
11. Mulkey, S. B., & du Plessis, A. J. (2019) Autonomic nervous system development and its impact on neuropsychiatric outcome. Pediatric Research 85, 120–126. doi:10.1038/s41390-018-0155-0
12. Scott-Solomon, E., Boehm, E. & Kuruvilla, R. (2021) The sympathetic nervous system in development and disease. Nature Reviews Neuroscience, 22, 685–702. doi:10.1038/s41583-02100523-y
13. Szolcsányi, J. (2015). Effect of capsaicin on thermoregulation: An update with new aspects. Temperature, 2(2), 277–296. doi:10.1080/2332894 0.2015.1048928
14. Kono, Y., Kubota, A., Taira, M., Katsuyama, N. & Sugimoto, K. (2018). Effects of oral stimulation with capsaicin on salivary secretion and neural activities in the autonomic system and the brain. Journal of Dental Sciences, 13(2), 116-123. doi:10.1016/j.jds.2017.08.007
15. Michlig, S., Merlini, J., Beaumont, M., Ledda, M., Tavenard, A., Mukherjee, R., Camacho, S., & le Coutre, J. (2016). Effects of TRP channel agonist ingestion on metabolism and autonomic nervous system in a randomized clinical trial of healthy subjects. Scientific Reports, 6. doi:10.1038/ srep20795
16. Gaiser, J., & Hayes, J. E. (2023). More than fat –Proteins in dairy and plant milks contribute to the reduction of oral burn from capsaicin. Food Quality and Preference, 112. doi:10.1016/j.foodqual.2023.105041
17. Nolden, A. A., Lenart, G., & Hayes, J. E. (2019). Putting out the fire - Efficacy of common beverages in reducing oral burn from capsaicin. Physiology & Behavior, 208. doi:10.1016/j.physbeh.2019.05.018
18. Farah, B. A., Hayes, J. E., & Coupland, J. N. (2022). The effect of dairy proteins on the oral burn of capsaicin. Journal of Food Science, 88(S1), A147-A157. doi:10.1111/1750-3841.16400
19. Choy, M., El Fassi, S., & Treur, J. (2021). An adaptive network model for pain and pleasure through spicy food and its desensitization. Cognitive Systems Research, 66, 211–220. doi:10.1016/j.cogsys.2020.10.006
20. Pilozzi, A., Carro, C., & Huang, X. (2021). Roles of β-endorphin in stress, behavior, neuroinflammation, and brain energy metabolism. International Journal of Molecular Sciences, 22(1). doi:10.3390/ ijms22010338
21. Aldhalemi, A. A., & Lahhob, Q. R. (2024). The study of chemical reactions in the human body through the use of hormones. International Journal of Advanced Biochemistry Research, 8(5), 1-9. doi:10.33545/26174693.2024.v8.i5a.1048
22. Fairbanks, C. A. & Peterson, C. D. (2023). The opioid receptor: Emergence through millennia of pharmaceutical sciences. Frontiers in Pain Research, 4. doi:10.3389/fpain.2023.960389
23. Smutzer, G. & Devassy, R. K. (2016). Integrating TRPV1 receptor function with capsaicin psychophysics. Advances in Pharmacological Sciences, 2016(1). doi:10.1155/2016/1512457
24. Berridge, K. C., & Robinson, T. E. (2016). Liking, wanting, and the incentive-sensitization theory of addiction. American Psychologist, 71(8), 670–679. doi:10.1037/amp0000059
25. Byrnes, N. K., & Hayes, J. E. (2015). Gender differences in the influence of personality traits on spicy food liking and intake. Food Quality and Preference, 42, 12–19. doi:10.1016/j.foodqual.2015.01.002
26. Byrnes, N. K., & Hayes, J. E. (2016). Behavioral measures of risk tasking, sensation seeking and sensitivity to reward may reflect different motivations for spicy food liking and consumption. Appetite, 103, 411–422. doi:10.1016/j.appet.2016.04.037
27. Spinelli, S., De Toffoli, A., Dinnella, C., Laureati, M., Pagliarini, E., Bendini, A., Braghieri, A., Gallina Toschi, T., Sinesio, F., Torri, L., Gasperi, F., Endrizzi, I., Magli, M., Borgogno, M., di Salvo, R., Favotto, S., Prescott, J., & Monteleone, E. (2018). Personality traits and gender influence liking and choice of food pungency. Food Quality and Preference, 66, 113–126. doi:10.1016/j.foodqual.2018.01.014
28. Siebert, E., Lee, S. Y., & Prescott, M. P. (2022). Chili pepper preference development and its impact on dietary intake: A narrative review. Frontiers in Nutrition, 9. doi:10.3389/ fnut.2022.1039207
29. Wang, X., Geng, L., Qin, J., & Yao, S. (2023). The potential relationship between spicy taste and risk seeking. Judgment and Decision Making, 11(6), 547–553. doi:10.1017/s1930297500004769
30. Nolden, A. A., Lenart, G., Spielman, A. I., & Hayes, J. E. (2024). Inducible desensitization to capsaicin with repeated low-dose exposure in human volunteers. Physiology & Behavior, 275. doi:10.1016/j.physbeh.2023.114447
31. Wang, S., Bian, C., Yang, J., Arora, V., Gao, Y., Wei, F., & Chung, M. K. (2020). Ablation of TRPV1+ afferent terminals by capsaicin mediates long-lasting analgesia for trigeminal neuropathic pain. eNeuro, 7(3). doi:10.1523/eneuro.0118-20.2020
32. Arora, V., Campbell, J. N., & Chung, M. K. (2020). Fight fire with fire: Neurobiology of capsaicin-induced analgesia for chronic pain. Pharmacology & Therapeutics, 220. doi:10.1016/j. pharmthera.2020.107743
33. Xiao, T., Sun, M., Zhao, C., & Kang, J. (2023). TRPV1: A promising therapeutic target for skin aging and inflammatory skin diseases. Frontiers in Pharmacology, 14. doi:10.3389/ fphar.2023.1037925
34. Derry, S., Rice, A. S., Cole, P., Tan, T., & Moore, R. A. (2017). Topical capsaicin (high concentration) for chronic neuropathic pain in adults. Cochrane Database of Systematic Reviews, 1(1). doi:10.1002/14651858.cd007393.pub4
35. Pathak, R., Sachan, N., & Chandra, P. (2022). Mechanistic approach towards diabetic neuropathy screening techniques and future challenges: A review. Biomedicine & Pharmacotherapy, 150. doi:10.1016/j.biopha.2022.113025
36. Weiss T, Koehler H, Croy I. (2022) Pain and reorganization after amputation: Is interoceptive prediction a key? The Neuroscientist, 29(6), 665675. doi:10.1177/10738584221112591
37. Kaur, A., & Guan, Y. (2018). Phantom limb pain: A literature review. Chinese Journal of Traumatology, 21(6), 366–368. doi:10.1016/j.cjtee.2018.04.006
38. Privitera, R., Birch, R., Sinisi, M., Mihaylov, I. R., Leech, R., & Anand, P. (2017). Capsaicin 8% patch treatment for amputation stump and phantom limb Pain: A clinical and functional MRI study. Journal of Pain Research, 10, 1623–1634. doi:10.2147/jpr.s140925
39. van Velzen, M., Dahan, A., & Niesters, M. (2020). Neuropathic pain: Challenges and opportunities. Frontiers in Pain Research, 1. doi:10.3389/ fpain.2020.00001
40. Goodwin, B., Maanas Chiplunkar, Salerno, R., Coombs, K., Umar Sannoh, Shah, V., Averell, N., Usmaan Al-Shebab, & Janora, D. M. (2023). Topical capsaicin for the management of painful diabetic neuropathy: A narrative systematic review. Pain Management, 13(5), 309–316. doi:10.2217/ pmt-2023-0006
41. Meacham K, Shepherd A, Mohapatra DP, Haroutounian S. (2017) Neuropathic pain: Central vs. peripheral mechanisms. Current Pain and Headache Reports, 21. doi:10.1007/s11916-017-0629-5
42. Cohen, K., Shinkazh, N., Frank, J., Israel, I., & Fellner, C. (2015). Pharmacological treatment of diabetic peripheral neuropathy. Pharmacy and Therapeutics, 40(6), 375-388. PMID:26045647
43. Obata, H. (2017). Analgesic mechanisms of antidepressants for neuropathic pain. International Journal of Molecular Sciences, 18(11). doi:10.3390/ijms18112483
44. Thouaye, M., & Yalcin, I. (2023). Neuropathic pain: From actual pharmacological treatments to new therapeutic horizons. Pharmacology & Therapeutics, 251. doi:10.1016/j.pharmthera.2023.108546
45. Jang, H. N., & Oh, T. J. (2023). Pharmacological and nonpharmacological treatments for painful diabetic peripheral neuropathy. Diabetes & Metabolism Journal, 47(6). doi:10.4093/ dmj.2023.0018
46. Simpson DM, Robinson-Papp J, Van J, Stoker M, Jacobs H, Snijder RJ, Schregardus DS, Long SK, Lambourg B, Katz N. (2017). Capsaicin 8% patch in painful diabetic peripheral neuropathy: A randomized, double-blind, placebo-controlled study. The Journal of Pain, 18(1), 42-53. doi:10.1016/j.jpain.2016.09.008
47. Landrum, O., Marcondes, L., Egharevba, T., & Gritsenko, K. (2023). Painful diabetic peripheral neuropathy of the feet: Integrating prescription-strength capsaicin into office procedures. Pain Management, 13(10), 613–626. doi:10.2217/ pmt-2023-0028
48. Alalami, K., Goff, J., Grimson, H., Martin, O., McDonald, E., Mirza, T., Mistry, D., Ofodile, A., Raja, S., Shaker, T., Sleibi, D., & Forget, P. (2024). Does topical capsaicin affect the central nervous system in neuropathic pain? A narrative review. Pharmaceuticals, 17(7), 842. doi:10.3390/ ph17070842
1. Iverson, G. L. (2015). Suicide and chronic traumatic encephalopathy. The Journal of Neuropsychiatry and Clinical Neurosciences, 28(1), 9–16. doi:10.1176/appi.neuropsych.15070172
2. De Souza Mendes Kawamura, L. R., Ferreira Lima Mota, I., Santos Vasconcelos, A., & Renata Mortari, M. (2023). Challenges in the pharmacological treatment of patients under suspicion of chronic traumatic encephalopathy: A review. Brain Research, 1799. doi:10.1016/j.brainres.2022.148176
3. McKee, A. C., Stein, T. D., Huber, B. R., Crary, J. F., Bieniek, K., Dickson, D., Alvarez, V. E., Cherry, J. D., Farrell, K., Butler, M., Uretsky, M., Abdolmohammadi, B., Alosco, M. L., Tripodis, Y., Mez, J., & Daneshvar, D. H. (2023). Chronic traumatic encephalopathy (CTE): Criteria for neuropathological diagnosis and relationship to repetitive head impacts. Acta Neuropathologica, 145(4), 371–394. doi:10.1007/s00401-023-02540-w
4. Alosco, M. L., Mian, A. Z., Buch, K., Farris, C. W., Uretsky, M., Tripodis, Y., Baucom, Z., Martin, B., Palmisano, J., Puzo, C., Ang, T. F. A., Joshi, P., Goldstein, L. E., Au, R., Katz, D. I., Dwyer, B., Daneshvar, D. H., Nowinski, C., Cantu, R. C., Kowall, N. W., Huber, B. R., Alvarez, V. E., Stern, R. A., Stein, T. D., Killiany, R. J., McKee, A. C., & Mez, J. (2021). Structural MRI profiles and tau correlates of atrophy in autopsy-confirmed CTE. Alzheimer’s Research & Therapy, 13(1), 193. doi:10.1186/ s13195-021-00928-y
5. Hollin, G. (2021). “Learning to listen to them and ask the right questions.” Bennet Omalu, scientific objectivities, and the witnessing of a concussion crisis. Frontiers in Sports and Active Living, 3. doi:10.3389/fspor.2021.672749
6. Robinson, R. M. (2024). Can probable trauma be compensated? The NFL’s CTE crisis and the ethics of compensating wages. In: Business Ethics: Kant, Virtue, and the Nexus of Duty (eds.) Springer. doi:10.1007/978-3-031-63122-1_13
7. Gerges, J.A., Chalhoub, I., Atallah, C., Khoury, R. (2023). Biomarkers of chronic traumatic encephalopathy: A state-of-the art review. Biomarkers in Neuropsychiatry, 8. doi:10.1016/j. bionps.2023.100066
8. LeClair, J., Weuve, J., Fox, M. P., Mez, J., Alosco, M. L., Nowinski, C., McKee, A., Yorghos, T. (2022). Relationship between level of American football playing and diagnosis of chronic traumatic encephalopathy in a selection bias analysis. American Journal of Epidemiology, 191(8), 1429-1443. doi:10.1093/aje/kwac075
9. Hageman, G., Hageman, I., Nihom, J. (2024). Chronic traumatic encephalopathy in soccer players: Review of 14 cases. Clinical Journal of Sport Medicine, 34(1), 69-80. doi:10.1097/ JSM.0000000000001174
10. VanItallie T. B. (2019). Traumatic brain injury (TBI) in collision sports: Possible mechanisms of transformation into chronic traumatic encephalopathy (CTE). Metabolism: Clinical and Experimental, 100. doi:10.1016/j.metabol.2019.07.007
11. Tribett, T., Erskine, B., Bailey, K., Brown, T., & Castellani, R. J. (2019). Chronic traumatic encephalopathy pathology after shotgun injury to the brain. Journal of Forensic Sciences, 64(4), 12481252. doi:10.1111/1556-4029.14001
12. Shively, S. B., Edgerton, S. L., Iacono, D., Purohit, D. P., Qu, B. X., Haroutunian, V., Davis, K. L., Diaz-Arrastia, R., & Perl, D. P. (2016). Localized cortical chronic traumatic encephalopathy pathology after single, severe axonal injury in human brain. Acta Neuropathologica, 133(3), 353–366. doi:10.1007/s00401-016-1649-7
13. Janković, T., & Pilipović, K. (2023). Single versus repetitive traumatic brain injury: Current knowledge on the chronic outcomes, neuropathology and the role of TDP-43 proteinopathy. Experimental Neurobiology, 32(4), 195–215. doi:10.5607/ en23008
14. Del Bigio, M. R., Krawitz, S., & Sinha, N. (2023). Chronic traumatic encephalopathy-neuropathologic change in a routine neuropathology service: 7-year follow-up. Journal of Neuropathology and Experimental Neurology, 82(11), 948–957. doi:10.1093/jnen/nlad079
15. Vink, R., & Corrigan, F. (2022). Chronic traumatic encephalopathy: Genes load the gun and repeated concussion pulls the trigger. Neural Regeneration Research, 17(9), 1963. doi:10.4103/16735374.335147
16. Feng, T., Lacrampe, A., & Hu, F. (2021). Physiological and pathological functions of TMEM106B: A gene associated with brain aging and multiple brain disorders. Acta Neuropathologica. doi:10.1007/s00401-020-02246-3
17. Jiao, H., Yuan, P., & Yu, J. (2023). TMEM106B aggregation in neurodegenerative diseases: Linking genetics to function. Molecular Neurodegeneration, 18(1). doi:10.1186/s13024-023-00644-1
18. Skaper, S. D., Facci, L., Zusso, M., & Giusti, P. (2018). An inflammation-centric view of neurological disease: Beyond the neuron. Frontiers in Cellular Neuroscience, 12. doi:10.3389/fncel.2018.00072
19. Udayar, V., Chen, Y., Sidransky, E., & Jagasia, R. (2022). Lysosomal dysfunction in neurodegeneration: emerging concepts and methods. Trends in Neurosciences, 45(3), 184–199. doi:10.1016/j. tins.2021.12.004
20. Cho, H., Hyeon, S. J., Shin, J. Y., Alvarez, V. E., Stein, T. D., Lee, J., Kowall, N. W., McKee, A. C., Ryu, H., & Seo, J. S. (2020). Alterations of transcriptome signatures in head trauma-related neurodegenerative disorders. Scientific Reports, 10(1). doi:10.1038/s41598-020-65916-y
21. Costello, K., & Greenwald, B. D. (2022). Update on domestic violence and traumatic brain injury: A narrative review. Brain Sciences, 12(1), 122. doi:10.3390/brainsci12010122
22. Iverson, G., & Gardner, A. J. (2021). Symptoms of traumatic encephalopathy syndrome are common in the United States general population. Brain Communications, 3(1). doi:10.1093/braincomms/fcab001
23. Gaetz, M. (2017). The multi-factorial origins of chronic traumatic encephalopathy (CTE) symptomology in post-career athletes: The athlete post-career adjustment (AP-CA) model. Medical Hypotheses, 102, 130–143. doi:10.1016/j. mehy.2017.03.023
24. Šimić, G., Tkalčić, M., Vukić, V., Mulc, D., Španić, E., Šagud, M., Olucha-Bordonau, F. E., Vukšić, M., & R. Hof, P. (2021). Understanding emotions: Origins and roles of the amygdala. Biomolecules, 11(6). doi:10.3390/biom11060823
25. Lepage, C., Muehlmann, M., Tripodis, Y., Hufschmidt, J., Stamm, J., Green, K., Wrobel, P., Schultz, V., Weir, I., Alosco, M. L., Baugh, C. M., Fritts, N. G., Martin, B. M., Chaisson, C., Coleman, M. J., Lin, A. P., Pasternak, O., Makris, N., Stern, R. A., & Shenton, M. E. (2018). Limbic system structure volumes and associated neurocognitive functioning in former NFL players. Brain Imaging and Behavior, 13(3), 725–734. doi:10.1007/s11682018-9895-z
26. Albayram, O., Albayram, S., & Mannix, R. (2020). Chronic traumatic encephalopathy—A blueprint for the bridge between neurological and psychiatric disorders. Translational Psychiatry, 10(1). doi:10.1038/s41398-020-01111-x
27. Mahar, I., Alosco, M. L., & McKee, A. C. (2017). Psychiatric phenotypes in chronic traumatic encephalopathy. Neuroscience & Biobehavioral Reviews, 83, 622–630. doi:10.1016/j.neubiorev.2017.08.023
28. Pîrşcoveanu, D. F. V., Pirici, I., Tudorică, V., Bălşeanu, T. A., Albu, V. C., Bondari, S., Bumbea, A. M., & Pîrşcoveanu, M. (2017). Tau protein in neurodegenerative diseases - A review. Romanian Journal of Morphology and Embryology, 58(4), 1141–1150. PMID:29556602
29. Logan, C. M., & Menko, A. S. (2019). Microtubules: Evolving roles and critical cellular interactions. Experimental Biology and Medicine, 244(15), 1240–1254. doi:10.1177/1535370219867296
30. Zhang, Y., Wu, K. M., Yang, L., Dong, Q., & Yu, J. T. (2022). Tauopathies: New perspectives and challenges. Molecular Neurodegeneration, 17, 28. doi:10.1186/s13024-022-00533-z
31. Benítez, M. J., Cuadros, R., & Jiménez, J. S. (2021). Phosphorylation and dephosphorylation of tau protein by the catalytic subunit of PKA, as probed by electrophoretic mobility retard. Journal of Alzheimer’s Disease, 79(3), 1143–1156. doi:10.3233/jad-201077
32. Wegmann, S., Biernat, J., & Mandelkow, E. (2021). A current view on tau protein phosphorylation in Alzheimer’s disease. Current Opinion in Neurobiology, 69, 131–138. doi:10.1016/j.conb.2021.03.003
33. Graham, N. S. N., Jolly, A., Zimmerman, K., Bourke, N. J., Scott, G., Cole, J. H., Schott, J. M., & Sharp, D. J. (2020). Diffuse axonal injury predicts neurodegeneration after moderate–severe traumatic brain injury. Brain, 143(12), 3685–3698. doi:10.1093/brain/awaa316
34. Zhang, X., Gao, F., Wang, D., Li, C., Fu, Y., He, W., & Zhang, J. (2018). Tau pathology in Parkinson’s disease. Frontiers in Neurology, 9. doi:10.3389/ fneur.2018.00809
35. Dixit, H., Kumar C, S., Chaudhary, R., Thaker, D., Gadewal, N., & Dasgupta, D. (2021). Role of phosphorylation and hyperphosphorylation of Tau in its interaction with Ba dimeric tubulin studied from a bioinformatics perspective. Avicenna Journal of Medical Biotechnology, 13(1), 24–34. doi:10.18502/ajmb.v13i1.4579
36. Moloney, C. M., Lowe, V. J., & Murray, M. E. (2021). Visualization of neurofibrillary tangle maturity in Alzheimer’s disease: A clinicopathologic perspective for biomarker research. Alzheimer’s & Dementia, 17(9), 1554-1574. doi:10.1002/alz.12321
37. Yang, J., Zhi, W., & Wang, L. (2024). Role of tau protein in neurodegenerative diseases and development of its targeted drugs: A literature review. Molecules, 29(12), 2812–2812. doi:10.3390/molecules29122812
38. Daneshvar, D. H., Goldstein, L. E., Kiernan, P. T., Stein, T. D., & McKee, A. C. (2015). Post-traumatic neurodegeneration and chronic traumatic encephalopathy. Molecular and Cellular Neuroscience, 66, 81–90. doi:10.1016/j.mcn.2015.03.007
39. Pan, L., Meng, L., He, M., & Zhang, Z. (2021). Tau in the pathophysiology of Parkinson’s disease. Journal of Molecular Neuroscience, 71(11), 21792191. doi:10.1007/s12031-020-01776-5
40. Blennow, K., & Zetterberg, H. (2018). Biomarkers for Alzheimer’s disease: Current status and prospects for the future. Journal of International Medicine, 284(6), 643-663. doi:10.1111/joim.12816
41. Gunes, S., Aizawa, Y., Sugashi, T., Sugimoto, M., & Rodrigues, P. P. (2022). Biomarkers for Alzheimer’s disease in the current state: A narrative review. International Journal of Molecular Sciences, 23(9), 4962. doi:10.3390/ijms23094962
42. Kim, N., Wang, B., Koikawa, K., Nezu, Y., Qiu, C., Lee, T. H., & Zhen, X. Z. (2021). Inhibition of death-associated protein kinase 1 attenuates cis p-tau and neurodegeneration in traumatic brain injury. Progress in Neurobiology, 203. doi:10.1016/j.pneurobio.2021.102072
43. Breen, P. W., & Krishnan, V. (2020). Recent preclinical insights into the treatment of chronic traumatic encephalopathy. Frontiers in Neuroscience, 14. doi:10.3389/fnins.2020.00616
44. Yadikar, H., Torres, I., Aiello, G., Kurup, M., Yang, Z., Lin, F., Kobeissy, F., Yost, R., & Wang, K. K. (2020). Screening of tau protein kinase inhibitors in a tauopathy-relevant cell-based model of tau hyperphosphorylation and oligomerization. PLOS One, 15(7). doi:10.1371/journal.pone.0224952
45. Arter, C., Trask, L., Ward, S., Yeoh, S., & Bayliss, R. (2022). Structural features of the protein kinase domain and targeted binding by small-molecule inhibitors. The Journal of Biological Chemistry, 298(8). doi:10.1016/j.jbc.2022.102247
46. Malhi, G. S., & Outhred, T. (2016). Therapeutic mechanisms of lithium in bipolar disorder: recent advances and current understanding. CNS Drugs, 30, 931–949. doi:10.1007/s40263-0160380-1
47. Sinnott, A. M., Chandler, M. C., Van Dyke, C., Mincberg, D. L., Pinapaka, H., Lauck, B. J., Mihalik, J. P. (2023). Efficacy of Guardian Cap soft-shell padding on head impact kinematics in American football: Pilot findings. International Journal of Environmental Research and Public Health, 20(21). doi:10.3390/ijerph20216991
48. Mills, S. & Canal, E. (2018). Prevention of athletic dental injuries: The mouthguard. Modern Sports Dentistry, 111-133. doi:10.1007/978-3-319-444161_7
49. Van Pelt, K. L., Caccese, J. B., Eckner, J. T., Putukian, M., Brooks, M. A., Cameron, K. L., Houston, M. N., Posner, M. A., Jackson, J. C., McGinty, G. T., Hillis, C. J., McAllister, T. W., McCrea, M. A., Broglio, S. P., & Buckley, T. A. (2021). Detailed description of Division I ice hockey concussions: Findings from the NCAA and Department of Defense CARE Consortium. Journal of Sport and Health Science, 10(2), 162–171. doi:10.1016/j. jshs.2021.01.004
50. Al Attar, W. S. A., Mahmoud, H., Alfadel, A., & Faude, O. (2024). Does headgear prevent sport-related concussion? A systematic review and meta-analysis of randomized controlled trials including 6311 players and 173,383 exposure hours. Sports Health, 16(3), 473–480. doi:10.1177/19417381231174461
51. Robinson, R. M. (2024). Can probable trauma be compensated? The NFL’s CTE crisis and the ethics of compensating wages. In: Business Ethics: Kant, Virtue, and the Nexus of Duty, 247-265. Springer. doi:10.1007/978-3-031-63122-1_13
52. Khan, S., & Talley, L. (2025). Beyond the hit: The hidden costs of repetitive head trauma. Neuroscience Insights, 20. doi: 10.1177/26331055251316315
53. Jacobi, J., Wasserman, E. B., D Mack, C., Heinzelmann, M., Cárdenas, J., Rehberg, R., Solomon, G., Sills, A., & Vargas, B. (2023). The National Football League concussion protocol: A review. HSS Journal, 19(3), 269–276. doi:10.1177/15563316231177207
54. Breedlove, K. M., Breedlove, E., Nauman, E., Bowman, T. G., & Lininger, M. R. (2017). The ability of an aftermarket helmet add-on device to reduce impact-force accelerations during drop tests. Journal of Athletic Training, 52(9), 802–808. doi:10.4085/1062-6050-52.6.01
55. Baker, H. P., Satinsky, A., Lee, C. S., Seidel, H., Dwyer, E., & Athiviraham, A. (2021). The targeting rule does not increase the rate of lower extremity injuries in NFL players over two seasons. The Physician and Sportsmedicine, 50(3), 239–243. doi:10.1080/00913847.2021.1910873
56. Concussions decrease to historic low in 2024 NFL season. (2025). NFL. Retrieved April 18, 2025 from operations.nfl.com/updates/football-ops/ concussions-decrease-to-historic-low-in-2024nfl-season/
57. Bieniek, K. F., Ross, O. A., Cormier, K. A., Walton, R. L., Soto-Ortolaza, A., Johnston, A. E., DeSaro, P., Boylan, K. B., Graff-Radford, N. R., Wszolek, Z. K., Rademakers, R., Boeve, B. F., McKee, A. C., & Dickson, D. W. (2015). Chronic traumatic encephalopathy pathology in a neurodegenerative disorders brain bank. Acta Neuropathologica, 130(6), 877–889. doi:10.1007/s00401-015-1502-4
10TH ANNIVERSARY OF GREY MATTERS JOUNRAL AT VASSAR COLLEGE: FACULTY PROFILES
DR.KATHLEENSUSMAN,PHD
1. Bradford, B. R., Whidden, E., Gervasio, E. D., Checchi, P. M., & Raley-Susman, K. M. (2020). Neonicotinoid-containing insecticide disruption of growth, locomotion, and fertility in Caenorhabditis elegans. PLOS ONE, 15(9), e0238637. doi:10.1371/journal.pone.0238637
DR.HADLEYBERGSTROM,PHD
1. Bergstrom, H. C., McDonald, C. G., Dey, S., Tang, H., Selwyn, R. G., & Johnson, L. R. (2013). The structure of Pavlovian fear conditioning in the amygdala. Brain Structure and Function, 218(6), 1569–1589. doi:10.1007/s00429-012-0478-2
2. Bergstrom, H. C., McDonald, C. G., Dey, S., Fernandez, G. M., & Johnson, L. R. (2013). Neurons activated during fear memory consolidation and reconsolidation are mapped to a common and new topography in the lateral amygdala. Brain Topography, 26(3), 468–478. doi:10.1007/s10548012-0266-6
3. Bergstrom, H. C., & Johnson, L. R. (2014). An organization of visual and auditory fear conditioning in the lateral amygdala. Neurobiologyof Learning and Memory, 116, 1–13. doi:10.1016/j. nlm.2014.07.008
4. McGuire, J. L., Bergstrom, H. C., Parker, C. C., Le, T., Morgan, M., Tang, H., Selwyn, R. G., Silva, A. C., Choi, K., Ursano, R. J., Palmer, A. A., & Johnson, L. R. (2013). Traits of fear resistance and susceptibility in an advanced intercross line. The European journal of neuroscience, 38(9), 3314–3324. https://doi.org/10.1111/ejn.12337
5. Bergstrom, H. C., Lipkin, A. M., Lieberman, A. G., Pinard, C. R., Gunduz-Cinar, O., Brockway, E. T., Taylor, W. W., Nonaka, M., Bukalo, O., Wills, T. A., Rubio, F. J., Li, X., Pickens, C. L., Winder, D. G., & Holmes, A. (2018). Dorsolateral Striatum Engagement Interferes with Early Discrimination Learning. Cell reports, 23(8), 2264–2272. https://doi. org/10.1016/j.celrep.2018.04.081
6. Subramanian, R., Bauman, A., Carpenter, O., Cho, C., Coste, G., Dam, A., Drake, K., Ehnstrom, S., Fitzgerald, N., Jenkins, A., Koolpe, H., Liu, R., Paserman, T., Petersen, D., Scala Chavez, D., Rozental, S., Thompson, H., Tsukuda, T., Zweig, S., Gall, M., Zupan, B., & Bergstrom, H. (2025). An infralimbic cortex engram encoded during learning attenuates fear generalization. Journal of Neuroscience. Advance online publication. doi:10.1523/ JNEUROSCI.2120-24.2025
DR.LORINEWMAN,PHD
1. Chiareli, R. A., Carvalho, G. A., Marques, B. L., Mota, L. S., Oliveira-Lima, O. C., Gomes, R. M., Birbrair, A., Gomez, R. S., Simão, F., Klempin, F., Leist, M., & Pinto, M. C. X. (2021). The Role of Astrocytes in the Neurorepair Process. Frontiers in cell and developmental biology, 9, 665795. doi:10.3389/ fcell.2021.665795
2. Mishra A. (2017). Binaural blood flow control by astrocytes: listening to synapses and the vasculature. The Journal of physiology, 595(6), 1885–1902. doi:10.1113/JP270979
3. Zhang, Y. M., Qi, Y. B., Gao, Y. N., Chen, W. G., Zhou, T., Zang, Y., & Li, J. (2023). Astrocyte metabolism and signaling pathways in the CNS. Frontiers in neuroscience, 17, 1217451. https://doi. org/10.3389/fnins.2023.1217451
4. Bélanger, M., & Magistretti, P. J. (2009). The role of astroglia in neuroprotection. Dialogues in clinical neuroscience, 11(3), 281–295. doi:10.31887/ DCNS.2009.11.3/mbelanger
5. Verkhratsky, A., Butt, A., Li, B., Illes, P., Zorec, R., Semyanov, A., Tang, Y., & Sofroniew, M. V. (2023). Astrocytes in human central nervous system diseases: a frontier for new therapies. Signal transduction and targeted therapy, 8(1), 396. doi:10.1038/s41392-023-01628-9
DR.JOHNLONG,PHD
1. Borrell, B. (2014). Why sharks have no bones. Nature. doi:10.1038/nature.2014.14487
DR.BOJANAZUPAN,PHD
1. Kietzman, H. W., & Gourley, S. L. (2023). How social information impacts action in rodents and humans: The role of the prefrontal cortex and its connections. Neuroscience and Biobehavioral Reviews, 147, 105075. doi:10.1016/j.neubiorev.2023.105075
2. Zupan, B., Sharma, A., Frazier, A., Klein, S., & Toth, M. (2016). Programming social behavior by the maternal fragile X protein. Genes, Brain and Behavior, 15(6), 578–587. doi:10.1111/gbb.12298
‘ONLY LOVE CAN HURT LIKE THIS’: UNDERSTANDING BROKEN HEART SYNDROME
1. Boyd, B., & Solh, T. (2020). Takotsubo cardiomyopathy. JAAPA, 33(3), 24–29. doi:10.1097/01. jaa.0000654368.35241.fc
2. Hansen, C., & Phillips, B. (2024a). ‘Wilt break my heart?’ Takotsubo syndrome and Shakespeare’s discourse of heartbreak in Antony and Cleopatra and King Lear. Shakespeare, 1–24. doi:10.1080/174 50918.2024.2319124
3. Wang, X., Pei, J., & Hu, X. (2020). The brainheart connection in Takotsubo syndrome: The central nervous system, sympathetic nervous system, and catecholamine overload. Cardiology Research and Practice, 2020(1), 1–5. doi:10.1155/2020/4150291
4. Ghadri, J. R., Wittstein, I. S., Prasad, A., Sharkey, S., Dote, K., Akashi, Y. J., Cammann, V. L., Crea, F., Galiuto, L., Desmet, W., Yoshida, T., Manfredini, R., Eitel, I., Kosuge, M., Nef, H. M., Deshmukh, A., Lerman, A., Bossone, E., Citro, R., Ueyyama, T., Corrado, D., Kurisu, S., Ruschitzka, F., Winchester, D., Lyon, A. R., Omerovic, E., Bax, J. J., Meimoun, P. Tarantini, G., Rihal, C., Hassan, S. Y., Migliore, F., Horowitz, J. D., Shimokawa, H., Lüscher, T. F., & Templin, C. (2018). International expert consensus document on Takotsubo syndrome (part I): Clinical characteristics, diagnostic criteria, and pathophysiology. European Heart Journal, 39(22), 2032–2046. doi:10.1093/eurheartj/ehy076
5. López Libano, J., Alomar Lladó, L., & Zarraga López, L. (2022). The Takotsubo syndrome: Clinical diagnosis using POCUS. POCUS Journal, 7(1), 137–139. doi:10.24908/pocus.v7i1.15296
6. Singh, T., Khan, H., Gamble, D. T., Scally, C., Newby, D. E., & Dawson, D. (2022). Takotsubo syndrome: Pathophysiology, emerging concepts, and clinical implications. Circulation, 145(13), 1002–1019. doi:10.1161/circulationaha.121.055854
7. Frank, N., Herrmann, M. J., Lauer, M., & Förster, C. Y. (2024). Exploratory review of the Takotsubo syndrome and the possible role of the psychosocial stress response and inflammaging. Biomolecules, 14(2), 167. doi:10.3390/biom14020167
8. Prasad, A., Lerman, A., & Rihal, C. S. (2008). Apical ballooning syndrome (tako-tsubo or stress cardiomyopathy): A mimic of acute myocardial infarction. American Heart Journal, 155(3), 408–417. doi:10.1016/j.ahj.2007.11.008
9. Templin, C., Napp, L. C., & Ghadri, J. R. (2016). Takotsubo syndrome: Underdiagnosed, underestimated, but understood? Journal of the American College of Cardiology, 67(16), 1937–1940. doi:10.1016/j.jacc.2016.03.006
10. Lu, L., Liu, M., Sun, R., Zheng, Y., & Zhang, P. (2015). Myocardial infarction: Symptoms and treatments. Cell Biochemistry and Biophysics, 72(3), 865–867. doi:10.1007/s12013-015-0553-4
11. Madias, J. E. (2021). Takotsubo cardiomyopathy: Current treatment. Journal of Clinical Medicine, 10(15), 3440. doi:10.3390/jcm10153440
12. Citro, R., Bellino, M., Merli, E., Di Vece, D., & Sherrid, M. V. (2023). Obstructive hypertrophic cardiomyopathy and Takotsubo syndrome: How to deal with left ventricular ballooning? Journal of the American Heart Association, 12(21). doi:10.1161/ jaha.123.032028
13. Awad, H. H., McNeal, A. R., & Goyal, H. (2018). Reverse Takotsubo cardiomyopathy: A comprehensive review. Annals of Translational Medicine, 6(23), 460. doi:10.21037/atm.2018.11.08
14. Lamotte, G., Shouman, K., & Benarroch, E. E. (2021). Stress and central autonomic network. Autonomic Neuroscience, 235, 102870. doi:10.1016/j.autneu.2021.102870
15. Hashemi, M. M., Gladwin, T. E., de Valk, N. M., Zhang, W., Kaldewaij, R., van Ast, V., Koch, S. B., Klumpers, F., & Roelofs, K. (2019). Neural dynamics of shooting decisions and the switch from freeze to fight. Scientific Reports, 9(1). doi:10.1038/s41598-019-40917-8
16. Ziemssen, T., & Siepmann, T. (2019b). The investigation of the cardiovascular and sudomotor autonomic nervous system—A review. Frontiers in Neurology, 10. doi:10.3389/fneur.2019.00053
17. Pelliccia, F., Kaski, J. C., & Camici, P. G. (2017). Pathophysiology of Takotsubo syndrome. American Heart Association Journal, 135(24). doi:10.1161/CIRCULATIONAHA.116.027121
18. Rotenberg, S., & McGrath, J. J. (2016). Inter-relation between autonomic and HPA axis activity in children and adolescents. Biological Psychology, 117, 16–25. doi:10.1016/j.biopsycho.2016.01.015
19. Murala, S., & Bollu, P. C. (2022). Norepinephrine. Neurochemistry in Clinical Practice, 165–179. doi:10.1007/978-3-031-07897-2_9
20. Gherasim, L., & Nistor, R. (2022). Neurogenic stunned myocardium as part of stress cardiomyopathy. Mædica, 17(4), 902–910. doi:10.26574/ maedica.2022.17.4.902
21. Y-Hassan, S. (2014). Acute cardiac sympathetic disruption in the pathogenesis of the Takotsubo syndrome: A systematic review of the literature to date. Cardiovascular Revascularization Medicine, 15(1), 35–42. doi:10.1016/j.carrev.2013.09.008
22. Amin, H. Z., Amin, L. Z., & Pradipta, A. (2020). Takotsubo cardiomyopathy: A brief review. Journal of Medicine and Life, 13(1), 3–7. doi:10.25122/ jml-2018-0067
23. Xiao, X., & Zhang, Y. (2018). A new perspective on the anterior cingulate cortex and affective pain. Neuroscience & Biobehavioral Reviews, 90, 200–211. doi:10.1016/j.neubiorev.2018.03.022
24. Brazdil, V., Kala, P., Hudec, M., Poloczek, M., Kanovsky, J., Stipal, R., Jerabek, P., Bocek, O., Pail, M., & Brazdil, M. (2022). The role of central autonomic nervous system dysfunction in Takotsubo syndrome: A systematic review. Clinical Autonomic Research, 32(1), 9–17. doi:10.1007/ s10286-021-00844-z
25. Silva, A. R., Magalhães, R., Arantes, C., Moreira, P. S., Rodrigues, M., Marques, P., Marques, J., Sousa, N., & Pereira, V. H. (2019). Brain functional connectivity is altered in patients with Takotsubo syndrome. Scientific Reports, 9(1). doi:10.1038/ s41598-019-40695-3
26. Osawa, A., Nagai, M., Dote, K., Kato, M., Oda, N., Kunita, E., Kagawa, E., Yamane, A., Kobatake, H., Shiota, H., Ishibashi, N., Takahashi, K., & Förster, C. Y. (2021). A mid-ventricular variant of Takotsubo syndrome: Was it triggered by insular cortex damage? ESC Heart Failure, 8(4), 3408–3412. doi:10.1002/ehf2.13397
27. Min, J., Farooq, M. U., Greenberg, E., Aloka, F., Bhatt, A., Kassab, M., Morgan, J. P., & Majid, A. (2010). Cardiac dysfunction after left permanent cerebral focal ischemia: The brain and heart connection. Stroke, 40(7), 2560–2563. doi:10.1161/ STROKEAHA.108.536086.
28. Radfar, A., Abohashem, S., Osborne, M. T., Wang, Y., Dar, T., Hassan, M. Z., Ghoneem, A., Naddaf, N., Patrich, T., Abbasi, T., Zureigat, H., Jaffer, J., Ghazi, P., Scott, J. A., Shin, L. M., Pitman, R. K., Neilan, T. G., Wood, M. J., & Tawakol, A. (2021). Stress-associated neurobiological activity associates with the risk for and timing of subsequent Takotsubo syndrome. European Heart Journal, 42(19), 1898–1908. doi:10.1093/eurheartj/ehab029
29. Khan, H., Gamble, D. T., Rudd, A., Mezincescu, A. M., Abbas, H., Noman, A., Stewart, A., Horgan, G., Krishnadas, R., Williams, C., Waiter, G. D., & Dawson, D. K. (2023). Structural and functional brain changes in acute Takotsubo syndrome. JACC: Heart Failure, 11(3), 307–317. doi:10.1016/j. jchf.2022.11.001
30. Šimić, G., Tkalčić, M., Vukić, V., Mulc, D., Španić, E., Šagud, M., Olucha-Bordonau, F. E., Vukšić, M., & R. Hof, P. (2021). Understanding emotions: Origins and roles of the amygdala. Biomolecules, 11(6), 823. doi:10.3390/biom11060823
31. Mohanty, R., Sethares, W. A., Nair, V. A., & Prabhakaran, V. (2020). Rethinking measures of functional connectivity via feature extraction. Scientific Reports, 10(1), 1298. doi:10.1038/s41598020-57915-w
32. Nyman, E., Mattsson, E., & Tornvall, P. (2019). Trigger factors in Takotsubo syndrome – A systematic review of case reports. European Journal of Internal Medicine, 63, 62–68. doi:10.1016/j. ejim.2019.02.017
33. Shakespeare, W., & Orgel, S. (ed.) (1999). King Lear. Penguin Books.
1. Tribelhorn, K., Twarużek, M., Kosicki, R., Straubinger, R. K., Ebel, F., & Ulrich, S. (2023). A chemically defined medium that supports mycotoxin production by Stachybotrys chartarum enabled analysis of the impact of nitrogen and carbon sources on the biosynthesis of macrocyclic trichothecenes and stachybotrylactam. Applied and Environmental Microbiology, 89(7). doi:10.1128/aem.00163-23
2. Lemons, A. R., Croston, T. L., Goldsmith, W. T., Barnes, M. A., Jaderson, M. A., Park, J. H., McKinney, W., Beezhold, D. H., & Green, B. J. (2019). Cultivation and aerosolization of Stachybotrys chartarum for modeling pulmonary inhalation exposure. International Forum for Respiratory Research, 31(13-14), 446-456.
3. Dyląg, M., Spychała, K., Zielinski, J., Łagowski, D., & Gnat, S. (2022). Update on Stachybotrys chartarum—Black mold perceived as toxigenic and potentially pathogenic to humans. Biology, 11(3), 352. doi:10.3390/biology11030352
4. Ibrahim, S. R. M., Choudhry, H., Asseri, A. H., Elfaky, M. A., Mohamed, S. G. A. & Mohamed, G. A. (2022). Stachybotrys chartarum—A hidden treasure: Secondary metabolites, bioactivities, and biotechnical relevance. Journal of Fungi, 8(5), 504. doi:10.3390/jof8050504
5. Höfte, H., & Voxeur, A. (2017). Plant cell walls. Current Biology, 27(17), R865-R870. doi:10.1016/j. cub.2017.05.025
6. Vance, P. H., Schaeffer, F., Terry, P., Trevino, E., & Weissfeld, A. S. (2016). Mold causes and effects “in a material world”. Clinical Microbiology Newsletter, 38(14), 111-116. doi:10.1016/j.clinmicnews.2016.06.004
7. Bava, R., Castagna, F., Piras, C., Musolino, V., Lupia, C., Palma, E., Britti, D., & Musella, V. (2022). Entomopathogenic fungi for pests and predators control in beekeeping. Veterinary Sciences, 9(2), 95. doi:10.3390/vetsci9020095
8. Oneto, D. L., Golan, J., Mazzino, A., Pringle, A., & Seminara, A. (2020). Timing of fungal spore release dictates survival during atmospheric transport. Proceedings of the National Academy of Sciences, 117(50), 5134-5143. doi:10.1073/ pnas.1913752117
9. Kraft, S., Buchenauer, L., & Polte, T. (2021). Mold, mycotoxins, and a dysregulated immune system: A combination of concern? International Journal of Molecular Sciences, 22(22). doi:10.3390/ ijms222212269
10. Croston, T. L., Lemons, A. R., Barnes, M. A., Goldsmith, W. T., Orandle, M. S., Nayak, A. P., Germolec, D. R., Green, B. J., & Beezhold, D. H. (2020). Inhalation of Stachybotrys chartarum fragments induces pulmonary arterial remodeling. American Journal of Respiratory Cell and Molecular Biology, 62(5), 563-576.
11. Omotayo, O. P., Omotayo, A. O., Mwanza, M., & Babalola, O. O. (2018). Prevalence of mycotoxins and their consequences on human health. Toxicological Research, 35(1), 1-7. doi:10.5487/ TR.2019.35.1.001
12. Zhao, Z., Zhang, Z., Zhang, H., & Liang, Z. (2022). Small peptides in the detection of mycotoxins and their potential applications in mycotoxin removal. Toxins, 14(11), 795. doi:10.3390/toxins14110795
13. McCarty, L. S., Borgert, C. J., & Burgoon, L. D. (2020). Evaluation of the inherent toxicity concept in environmental toxicology and risk assessment. Environmental Toxicology and Chemistry, 39(12), 2351-2360. doi:10.1002/etc.4881
14. Marcelloni, A. M., Pigini, D., Chiominto, A., Gioffrè, A., & Paba, E. (2023). Exposure to airborne mycotoxins: The riskiest working environments and tasks. Annals of World Exposures and Health, 68(1), 19-35. doi:10.1093/annweh/wxad070
15. Scherzad, A., Hagen, R., & Hackenberg, S. (2019). Current understanding of nasal epithelial cell mis-differentiation. Journal of Inflammation Research, 12, 309-317. doi:10.2147/JIR.S180853
16. Choi, R., & Goldstein, B. J. (2018). Olfactory epithelium: Cells, clinical disorders, and insights from an adult stem cell niche. Laryngoscope Investigative Otolaryngology, 3(1), 35-42. doi:10.1002/lio2.135
17. Middleton, S. J., Perez-Sanchez, J., & Dawes, J. M. (2022). The structure of sensory afferent compartments in health and disease. Journal of Anatomy, 241(5), 1186-1210. doi:10.1111/joa.13544
18. Omura, K., Han, B., Nishijima, H., Aoki, S., Ebihara, T., Kondo, K., Otori, N., Kojima, H., Yamasoba, T., & Kikuta, S. (2021). Heterogeneous distribution of mature olfactory sensory neurons in human olfactory epithelium. International Forum of Allergy & Rhinology, 12(3), 266-277. doi:10.1002/alr.22885
19. Hiranuma, M., Okuda, Y., Fujii, Y., Richard, J. P., & Watanabe, T. (2024). Characterization of human iPSC-derived sensory neurons and their functional assessment using multi electrode array. Scientific Reports, 14. doi:10.1038/s41598-02455602-8
20. Ehsanifar, M., Rajati, R., Gholami, A., & reiss, J. P. (2023). Mold and mycotoxin exposure and brain disorders. Journal of Integrative Neuroscience, 22(6), 137. doi:10.31083/j.jin2206137
21. Chaudhary, Z., Rehman, K., & Akash M. S. H. (2021). Mechanistic insight of myotoxin-induced neurological disorders and treatment strategies. In: Akash, M. S. H., & Rehman, K. (eds.) Environmental Contaminants and Neurological Disorders. Emerging Contaminants and Associated Treatment Technologies. Springer. doi:10.1007/978-3-030-66376-6_7
22. Mavrommatis, A., Giamouri, E., Tavrizelou, S., Zacharioudaki, M., Danezis, G., Simitzis, P. E., Zoidis, E., Tsiplakou, E., Pappas, A. C., Georgiou, C. A., & Feggeros, K. (2021). Impact of mycotoxins on animals’ oxidative status. Antioxidants, 10(2). doi:10.3390/antiox10020214
23. Cleary, D. (2022). Effects of mycotoxins on macrophages and their possible clinical implications. In: Gupta. S., & Pathak, Y. V. (eds.) Macrophage Targeted Delivery Systems. Springer. doi:10.1007/978-3-030-84164-5_23
24. Chandimali, N., Bak, S. G., Park, E. H., Lim, H. J., Won, Y. S., Kim, E. K., Park, S. I., & Lee, S. J. (2025). Free radicals and their impact on health and antioxidant defenses: A review. Cell Death Discovery, 11. doi:10.1038/s41420-024-02278-8
25. Tan, B. L., Norhaizan, M. E., Liew, W. P. P., & Rahman, H. S. (2018). Antioxidant and oxidative stress: A mutual interplay in age-related diseases. Frontiers in Pharmacology, 9. doi:10.3389/ fphar.2018.01162
26. Hassan, H. A., Ahmed, H. S., & Hassan, D. F. (2024). Free radicals and oxidative stress: Mechanisms and therapeutic targets. Human antibodies, 32(4), 151-167. doi:10.3233/HAB-240011
27. Kumar, R. R., Arora, K., Goswami, S., Sakhare, A., Singh, B., Chinnusamy, V., & Praveen, S. (2020). MAPK enzymes: A ROS activated signaling sensors involved in modulating heat stress response, tolerance and grain stability of wheat under stress. 3 Biotech, 10(9). doi:10.1007/s13205020-02377-0
28. Chen, M. J., Ramesha, S., Weinstock, L. D., Gao, T., Ping, L., Xiao, H., Dammer, E. B., Duong, D. D., Levey, A. I., Lah, J. J., Seyfried, N. T., Wood, L. B., & Rangaraju, S. (2021). Inflammatory responses and inflammation-associated diseases in organs. Journal of Neuroscience Research, 99(6), 17041721. doi:10.1002/jnr.24829
29. Shao, F., Wang, X., Wu, H., Wu, Q., & Zhang, J. (2022). Microglia and neuroinflammation: Crucial pathological mechanisms in traumatic brain injury-induced neurodegeneration. Frontiers in Aging Neuroscience, 14. doi:10.3389/fnagi.2022.825086
30. Ferro, A., Aguste, Y. S., S., & Cheadle, L. (2021). Microglia, cytokines, and neural activity: Unexpected interactions in brain development and function. Frontiers in Immunology, 12. doi:10.3389/fimmu.2021.703527
31. Ishihara, Y., Itoh, K. (2022). Microglial inflammatory reactions regulated by oxidative stress. Journal of Clinical Biochemistry and Nutrition, 72(1), 23-27. doi:10.3164/jcbn.22-71
32. Vervaeke, A., & Lamkanfi, M. (2025). MAP kinase signaling at the crossroads of inflammasome activation. Immunological reviews, 329(1). doi:10.1111/imr.13436
33. LaFever, B. J., & Imamura, F. (2022). Effects of nasal inflammation on the olfactory bulb. Journal of Neuroinflammation, 9(19). doi:10.1186/ s12974-022-02657-x
34. Kulcsár, S., Kövesi, B., Balogh, K., Zándoki, E., Ancsin, Z., Erdélyi, M. & Mézes, M. (2023). The co-occurrence of T-2 toxin, deoxynivalenol, and fumonisin B1 activated the glutathione redox system in the EU-limiting doses in laying hens. Toxins, 15(5). doi:10.3390/toxins15050305
35. Hurraß, J., Nowak, D., Heinzow, B., Joest, M., Stemler, J., & Wiesmüller, G. A. (2024). Indoor mold. Deutches Ärtzeblatt, 121, 265-271. doi:10.3238/arztebl.m2024.0018
36. Rea, W. J. (2018). A large case-series of successful treatment of patients exposed to mold and mycotoxin. Clinical Therapeutics, 40(6), 889-893. doi:10.1016/j.clinthera.2018.05.003
37. Nag, P. K. (2018): Sick building syndrome and other building-related illnesses. Office Buildings, 53-103. doi:10.1007/978-981-13-2577-9_3
38. Subri, M. S. M., Arifin, K., Sohaimin, M. F. A. M., & Abas, A. (2024). The parameter of the sick building syndrome: A systematic literature review. Heliyon, 10(12). doi:10.1016/j.heliyon.2024.e32431
39. Mansor, A. A., Abdullah, S., Ahmad, A. N., Ahmed, A. N., Zulkifli, M. F. R., Jusoh, S. M., & Ismail, M. (2024). Indoor air quality and sick building syndrome symptoms in administrative office at public university. Dialogues in Health, 4. doi:10.1016/j. dialog.2024.100178
40. Lu, C., Deng, Q., Li, Y., Sundell, J., & Norbäck, D. (2016). Outdoor air pollution, meteorological conditions and indoor factors in dwellings in relation to sick building syndrome (SBS) among adults in China. Science of the Total Environment, 560561, 186–196. doi:10.1016/j.scitotenv.2016.04.033
41. U.S. Department of Health and Human Services National Toxicology Program (2024). NTP technical report on the toxicity study of Stachybotrys chartarum (CASRN 67892-26-6) administered by inhalation to B6C3F1/N mice. Retrieved from https://ntp.niehs.nih.gov/sites/default/ files/2024-09/tox107_508.pdf
42. Harding, C. Ff., Pytte, C. L., Page, K. G., Ryberg, K. J., Normand, E., Remigio, G. J., DeStefano, R. A., Morris, D. B., Voronina, J., Lopez, A., Stalbow, L. A., Williams, E. P., & Abreu, H. (2021). Mold inhalation causes innate immune activation, neural, cognitive, and emotional dysfunction. Brain, Behavior, and Immunity, 87, 218-228. doi:10.1016/j. bbi.2019.11.006
1. Archibald, L. M. (2017). SLP-educator classroom collaboration: A review to inform reason-based practice. Autism & Developmental Language Impairments, 2. doi:10.1177/2396941516680369
2. MacNeill, K. (2021). Communication Disorder/ Communication Impairment. In: Volkmar, F. R. (eds.) Encyclopedia of Autism Spectrum Disorders. Springer, Cham. doi:10.1007/978-3-31991280-6_766
3. Brito-Marcelino, A., Oliva-Costa, E. F., Sarmento, S. C., & Carvalho, A. A. (2020). Burnout syndrome in speech-language pathologists and audiologists: A review. Revista Brasileira de Medicina Do Trabalho, 18(2). doi:10.47626/1679-4435-2020480
4. Godoy, J., Silverio, K., & Brasolotto, A. (2019). Effectiveness of vocal therapy for the elderly when applying conventional and intensive approaches: A randomized clinical trial. Journal of Voice, 33(5). doi:10.1016/j.jvoice.2018.03.017
5. Ross, K., Heiny, E., Conner, S., Spener, P., & Pineda, R. (2017). Occupational therapy, physical therapy and speech-language pathology in the neonatal intensive care unit: Patterns of therapy usage in a level IV NICU. Research in Developmental Disabilities, 64, 108–117. doi:10.1016/j. ridd.2017.03.009
6. Farquharson, K., Therrien, M., Barton-Hulsey, A., & Brandt, A. F. (2020). How to recruit, support, and retain speech-language pathologists in public schools. Journal of School Leadership, 32(3), 225–245. doi:10.1177/1052684620966062
7. Burns, J., Keaveny, C., Nieto, N., O’Connor, R., & Moss, H. (2024). Collaborative music therapy and speech-language pathology for pediatric acquired communication impairments: A phenomenological international perspective. American Music Therapy Association, 43(1). doi:10.1093/ mtp/miae026
8. Huang, A. J., Siyambalapitiya, S., & Cornwell, P. (2019). Speech pathologists and professional interpreters managing culturally and linguistically diverse adults with communication disorders: A systematic review. International Journal of Language & Communication Disorders, 54(5), 689–704. doi:10.1111/1460-6984.12475
9. Nuara, A. (2020). Music and mirror neuron system. In: Colombo, B. (eds.) Brain and Art. Springer. doi:10.1007/978-3-030-23580-2_7
10. Haro-Martínez, A., Pérez-Araujo, C. M., Sanchez-Caro, J. M., Fuentes, B., & Díez-Tejedor, E. (2021). Melodic intonation therapy for poststroke non-fluent aphasia: Systematic Review and meta-analysis. Frontiers in Neurology, 12. doi:10.3389/fneur.2021.700115
11. Ong, A., Namasivayam-MacDonald, A., Kim, S., & Werden Abrams, S. (2024). The use of music and music-related elements in speech-language therapy interventions for adults with neurogenic communication impairments: A scoping review. International Journal of Language & Communication Disorders, 59(6), 2632-2654. doi:10.1111/14606984.13104
12. Irons, J. Y., Hancox, G., Vella-Burrows, T., Han, E. Y., Chong, H. J., Sheffield, D., & Stewart, D. E. (2020). Group singing improves quality of life for people with Parkinson’s: An international study. Aging & Mental Health, 25(4), 650–656. doi:10.108 0/13607863.2020.1720599
13. Monroe, P., Halaki, M., Kumfor, F., & Ballard, K. J. (2020). The effects of choral singing on communication impairments in acquired brain injury: A systematic review. International Journal of Language & Communication Disorders, 55(3), 303–319. doi:10.1111/1460-6984.12527
14. Chorna, O., Filippa, M., De Almeida, J. S., Lordier, L., Monaci, M. G., Hüppi, P., Grandjean, D., & Guzzetta, A. (2019). Neuroprocessing mechanisms of music during fetal and neonatal development: A role in neuroplasticity and Neurodevelopment. Neural Plasticity, 2019(1), 1–9. doi:10.1155/2019/3972918
15. Fiveash, A., Bedoin, N., & Tillmann, B. (2024). Examining methodological influences on the rhythmic priming effect: A commentary on Kim, McLaren, and Lee. Journal of Experimental Child Psychology, 250. doi:10.1016/j.jecp.2024.106111
16. Virtala, P., & Partanen, E. (2018). Can very early music interventions promote at risk infants’ development? Annals of the New York Academy of Sciences, 1423(1), 92–101. doi:10.1111/nyas.13646
17. Arenillas-Alcon, S., Ribas-Prats, T., Puertollano, M., Mondejar-Segovia, A., Dolores Gomez-Roig, M., Costa-Faidella, J., & Escera, C. (2022). Prenatal daily musical exposure is associated with enhanced neural representation of speech fundamental frequency: Evidence from neonatal frequency-following responses. Developmental Science, 26(5). doi:10.1111/desc.13362
18. Asano, R., & Boeckx, C. (2015). Syntax in language and music: What is the right level of comparison? Frontiers in Psychology, 6. doi:10.3389/ fpsyg.2015.00942
19. Stadler Elmer, S. (2020). From canonical babbling to early singing and its relation to the beginnings of speech. In: Russo, F. A., Ilari, B., & Cohen, A. J. (eds.). The Routledge Companion to Interdisciplinary Studies in Singing, 25–38. Routledge. doi:10.4324/9781315163734-2
20. Vuust, P., Heggli, O.A., Friston, K.J., & Kringelbach, M. L. (2022). Music in the brain. Nature Reviews Neuroscience, 23, 287–305. doi:10.1038/s41583022-00578-5
21. Brown, S. (2017). A joint prosodic origin of language and music. Frontiers in Psychology, 8. doi:10.3389/fpsyg.2017.01894
22. Friederici, A. D. (2018). The neural basis for human syntax: Broca’s area and beyond. Current Opinion in Behavioral Sciences, 21, 88–92. doi:10.1016/j.cobeha.2018.03.004
23. Kunert, R., Willems, R. M., Cassanto, D., Patel, A. D., & Hagroot, P. (2015). Music and language syntax interact in Broca’s area: An fMRI study. PLOS One, 10(11). doi:10.1371/journal.pone.0141069
24. Slater, J., Skoe, E., Strait, D. L., O’Connell, S., Thompson, E., & Kraus, N. (2015). Music training improves speech-in-noise perception: Longitudinal evidence from a community-based music program. Behavioural Brain Research, 291, 244–252. doi:10.1016/j.bbr.2015.05.026
25. Falk, S., & Kello, C. T. (2017). Hierarchical organization in the temporal structure of infant-direct speech and song. Cognition, 163, 80–86. doi:10.1016/j.cognition.2017.02.017
26. Sallat, S., & Jentschke, S. (2015). Music perception influences language acquisition: Melodic and rhythmic-melodic perception in children with specific language impairment. Behavioural Neurology, 2015, 1–10. doi:10.1155/2015/606470
27. Waring, R., Rickard Liow, D., Eadie, P., & Dobb, B.(2019). Speech development in preschool children: Evaluating the contribution of phonological short-term and phonological working memory. Journal of Child Language, 46(4), 632–652. doi:10.1017/s0305000919000035
28. Chern, A., Tillmann, B., Vaughan, C., & Gordon, R. L. (2018). New evidence of a rhythmic priming effect that enhances grammaticality judgments in children. Journal of Experimental Child Psychology, 173, 371–379. doi:10.1016/j.jecp.2018.04.007
29. Cason, N., Astésano, C., & Schön, D. (2015). Bridging Music and speech rhythm: Rhythmic priming and audio–motor training affect speech perception. Acta Psychologica, 155, 43–50. doi:10.1016/j. actpsy.2014.12.002
30. Roberts, J. A., Gollo, L. L., Abeysuriya, R. G., Roberts, G., Mitchell, P. B., Woolrich, M. W., & Breakspear, M. (2019). Metastable brain waves. Nature Communications, 10(1). doi:10.1038/s41467-01908999-0
31. Zhao, C. T., & Kuhl, P. K. (2016). Musical intervention enhances infants’ neural processing of temporal structure in music and speech. Proceedings of the National Academy of Science in the United States of America, 113(19), 5212-5217. doi:10.1073/pnas.1603984113
32. Papatzikis, E., & Rishony, H. (2022). What is music for neuroplasticity? Combined value on infant development and inclusion. In: Efstratopoulou, M. (eds.). Rethinking Inclusion and Transformation in Special Education, 160–177. IGI Global. doi:10.4018/978-1-6684-4680-5.ch010
33. Xing, S., Lacey, E. H., Skipper-Kallal, L. M., Jiang, X., Harris-Love, M. L., Zeng, J., & Turkeltaub, P. E. (2015). Right hemisphere grey matter structure and language outcomes in chronic left hemisphere stroke. Brain, 139(1), 227–241. doi:10.1093/ brain/awv323
34. Hartshorne, J. K., Tenenbaum, J. B., & Pinker, S. (2018). A critical period for second language acquisition: Evidence from 2/3 million English speakers. Cognition, 177, 263–277. doi:10.1016/j. cognition.2018.04.007
35. Heyes, C., & Catmur, C. (2021). What happened to mirror neurons? Perspectives on Psychological Science, 17(1), 153–168. doi:10.1177/1745691621990638
36. Ryan, A., Gibbon, F. E., & O’shea, A. (2015). Expressive and receptive language skills in preschool children from a socially disadvantaged area. International Journal of Speech-Language Pathology, 18(1), 41–52. doi:10.3109/17549507.2015 .1089935
37. Chatterjee, D., Hegde, S., & Thaut, M. (2021). Neural plasticity: The substratum of music-based interventions in neurorehabilitation. NeuroRehabilitation, 48(2), 155–166. doi:10.3233/nre-208011
38. Proverbio, A. M., & Piotti, E. (2021). Common neural bases for processing speech prosody and music: An integrated model. Psychology of Music, 50(5), 1408–1423. doi:10.1177/03057356211050117
39. Zhang, X., Li, J., & Du, Y. (2022). Melodic intonation therapy on non-fluent aphasia after stroke: A systematic review and analysis on clinical trials. Frontiers in Neuroscience, 15. doi:10.3389/ fnins.2021.753356
40. Bharathi, G., Jayaramayya, K., Balasubramanian, V., & Vellingiri, B. (2019). The potential role of rhythmic entrainment and music therapy intervention for individuals with autism spectrum disorders. Journal of Exercise Rehabilitation, 15(2), 180–186. doi:10.12965/jer.1836578.289
41. Loomes, R., Hull, L., & Mandy, W. P. (2017). What is the male-to-female ratio in autism spectrum disorder? A systematic review and meta-analysis. Journal of the American Academy of Child & Adolescent Psychiatry, 56(6), 466–474. doi:10.1016/j. jaac.2017.03.013
42. Shi, Z., Wang, S., Chen, M., Hu, A., Long, Q., & Lee, Y. (2024). The effect of music therapy on language communication and social skills in children with autism spectrum disorder: A systematic review and meta-analysis. Frontiers in Psychology, 15. doi:10.3389/fpsyg.2024.1336421
43. Ardila, A., Bernal, B., & Rosselli, M. (2016). How extended is Wernicke’s area? Meta-analytic connectivity study of BA20 and integrative proposal. Neuroscience Journal, 2016, 1–6. doi:10.1155/2016/4962562
44. Lee, Y., Park, B., James, O., Kim, S. G., & Park, H. (2017). Autism spectrum disorder related functional connectivity changes in the language network in children, adolescents and adults. Frontiers in Human Neuroscience, 11. doi:10.3389/ fnhum.2017.00418
45. Bastos, A. M., & Schoffelen, J. M. (2016). A tutorial review of functional connectivity analysis methods and their interpretational pitfalls. Frontiers in Systems Neuroscience, 9. doi:10.3389/fnsys.2015.00175
46. Long, Z., Duan, X., Mantini, D., & Chen, H. (2016). Alteration of functional connectivity in autism spectrum disorder: Effect of age and anatomical distance. Scientific Reports, 6(1). doi:10.1038/ srep26527
47. Snyder, W., & Troiani, V. (2020). Behavioural profiling of autism connectivity abnormalities. BJPsych Open, 6(1). doi:10.1192/bjo.2019.102
48. Chenausky, K., Norton, A., Tager-Flusberg, H., & Schlaug, G. (2016). Auditory-motor mapping training: Comparing the effects of a novel speech treatment to a control treatment for minimally verbal children with autism. PLOS One, 11(11). doi:10.1371/journal.pone.0164930
49. Leadbitter, K., Buckle, K. L., Ellis, C., & Dekker, M. (2021). Autistic self-advocacy and the neurodiversity movement: Implications for autism early intervention research and practice. Frontiers in Psychology, 12. doi:10.3389/fpsyg.2021.635690
50. Ahlers, K. P., Gabrielsen, T. P., Lewis, D., Brady, A. M., & Litchford, A. (2017). Supporting individuals with autism spectrum disorder in understanding and coping with complex social emotional issues. School Psychology International, 38(6), 586–607. doi:10.1177/0143034317719942
51. Lamm, C., & Majdandžić, J. (2015). The role of shared neural activations, mirror neurons, and morality in empathy – A critical comment. Neuroscience Research, 90, 15–24. doi:10.1016/j. neures.2014.10.008
52. Chan, M. M. Y., & Han, Y. M. Y. (2020). Differential mirror neuron system (MNS) activation during action observation with and without social-emotional components in autism: A meta-analysis of neuroimaging studies. Molecular Autism, 11. doi:10.1186/s13229-020-00374-x
53. Morales, M., & Margolis, E. B. (2017). Ventral tegmental area: Cellular heterogeneity, connectivity and behaviour. Nature Reviews Neuroscience, 18(2), 73–85. doi:10.1038/nrn.2016.165
54. Berke, J. D. (2018). What does dopamine mean? Nature Neuroscience, 21(6), 787–793. doi:10.1038/ s41593-018-0152-y
55. Goldman, J. G., Vernaleo, B. A., Camicioli, R., Dahodwala, N., Dobkin, R. D., Ellis, T., Galvin, J. E., Marras, C., Edwards, J., Fields, J., Golden, R., Karlawish, J., Levin, B., Shulman, L., Smith, G., Tangney, C., Thomas, C. A., Tröster, A. I., Uc, E. Y., Coyan, N., Ellman, C., Ellman, M., Hoffman, C., Hoffman, S., & Simmonds, D. (2018). Cognitive impairment in Parkinson’s disease: A report from a multidisciplinary symposium on unmet needs and future directions to maintain cognitive health. NPJ Parkinson’s Disease, 4(1). doi:10.1038/ s41531-018-0055-3
56. Fereshtehnejad, S. M., & Postuma, R. B. (2017). Subtypes of Parkinson’s disease: What do they tell us about disease progression? Current Neurology and Neuroscience Reports, 17(4). doi:10.1007/s11910-017-0738-x
57. Pylypowich, A., & Duff, E. (2016). Differentiating the symptom of dysphonia. The Journal for Nurse Practitioners, 12(7), 459–466. doi:10.1016/j. nurpra.2016.04.025
58. Stachler, R. J., Francis, D. O., Schwartz, S. R., Damask, C. C., Digoy, G. P., Krouse, H. J., McCoy, S. J., Ouellette, D. R., Patel, R. R., Reavis, C. (Charlie), Smith, L. J., Smith, M., Strode, S. W., Woo, P., & Nnacheta, L. C. (2018). Clinical practice guideline: Hoarseness (dysphonia) (update). Otolaryngology–Head and Neck Surgery, 158(S1). doi:10.1177/0194599817751030
59. Rampello, L., Patti, F., & Zappia, M. (2016). When the word doesn’t come out: A synthetic overview of dysarthria. Journal of the Neurological Sciences, 369, 354–360. doi:10.1016/j.jns.2016.08.048
60. Tamplin, J., Morris, M. E., Marigliani, C., Baker, F. A., & Vogel, A. P. (2019). ParkinSong: A controlled trial of singing-based therapy for Parkinson’s disease. Neurorehabilitation and Neural Repair, 33(6), 453–463. doi:10.1177/1545968319847948
61. Kang, J., Scholp, A., & Jiang, J. J. (2018). A review of the physiological effects and mechanisms of singing. Journal of Voice, 32(4), 390–395. doi:10.1016/j.jvoice.2017.07.008
62. T., Zaatar, M., Alhakim, K., Enayeh, M., & Tamer, R. (2024). The transformative power of music: Insights into neuroplasticity, health, and disease. Brain, Behavior, & Immunity - Health, 35. doi:10.1016/j.bbih.2023.100716
1. Malgaroli, M., Calderon, A., & Bonanno, G. A., (2021). Networks of major depressive disorder: A systematic review. Clinical Psychology Review, 85. doi:10.1016/j.cpr.2021.102000
2. Skaper, S. D., Facci, L., Zusso, M., & Giusti, P. (2018). An inflammation-centric view of neurological disease: Beyond the neuron. Frontiers in Cellular Neuroscience, 12. doi:10.3389/fncel.2018.00072
3. Remes, O., Mendes, J. F., & Templeton, P. (2021). Biological, psychological, and social determinants of depression: A review of recent literature. Brain Sciences, 11(12). doi:10.3390/brainsci11121633
4. Yirmiya, R. (2024). The inflammatory underpinning of depression: A historical perspective. Brain, Behavior, and Immunity, 122, 433-443. doi:10.1016/j.bbi.2024.08.048
5. Chen, L., Deng, H., Cui, H., Fang, J., Zuo, Z., Deng, J., Li, Y., Wang, X., & Zhao, L. (2017). Inflammatory responses and inflammation-associated diseases in organs. Oncotarget, 9(6), 7204–7218. doi:10.18632/oncotarget.23208
6. Gaudet, A. D., & Fonken, L. K. (2018). Glial cells shape pathology and repair after spinal cord injury. Neurotherapeutics 15, 554–577. doi:10.1007/ s13311-018-0630-7
7. Gomez-Nicola, D., & Perry, V. H. (2015). Microglial dynamics and role in the healthy and diseased brain. The Neuroscientist, 21(2), 169–184. doi:10.1177/1073858414530512
8. Augusto-Oliveira, M., Arrifano, G. P., Takeda, P. Y., Lopes-Araújo, A., Santos-Sacramento, L., Anthony, D. C., Verkhratsky, A., & Crespo-Lopez, M. E. (2020). Astroglia-specific contributions to the regulation of synapses, cognition and behaviour. Neuroscience & Biobehavioral Reviews, 118, 331–357. doi:10.1016/j.neubiorev.2020.07.039
9. Baldwin, K. T., Murai, K. K., & Khakh, B. S. (2024). Astrocyte morphology. Trends in Cell Biology, 34(7), 547–565. doi:10.1016/j.tcb.2023.09.006
10. Siracusa, R., Fusco, R., & Cuzzocrea, S. (2019). Astrocytes: Role and functions in brain pathologies. Frontiers in Pharmacology, 10. doi:10.3389/ fphar.2019.01114
11. Colonna, M., & Butovsky, O. (2017). Microglia function in the central nervous system during health and neurodegeneration. Annual Review of Immunology, 35, 441–468. doi:10.1146/annurev-immunol-051116-052358
12. Lawrence, J. M., Schardien, K., Wigdahl, B., & Nonnemacher, M. R. (2023). Roles of neuropathology-associated reactive astrocytes: A systematic review. Acta Neuropathologica Communications, 11. doi:10.1186/s40478-023-01526-9
13. Gao, C., Jiang, J., Tan, Y., & Chen, S. (2023). Microglia in neurodegenerative diseases: Mechanism and potential therapeutic targets. Signal Transduction and Targeted Therapy, 8. doi:10.1038/s41392-023-01588-0
14. Moyse, E., Krantic, S., Djellouli, N., Roger, S., Angoulvant, D., Debacq, C., Leroy, V., Fougere, B., & Aidoud, A. (2022). Neuroinflammation: A possible link between chronic vascular disorders and neurodegenerative diseases. Frontiers in Aging Neuroscience, 14. doi:10.3389/fnagi.2022.827263
15. Homem, C. C., Repic, M., & Knoblich, J. A. (2015). Proliferation control in neural stem and progenitor cells. Nature Reviews Neuroscience, 16(11), 647–659. doi:10.1038/nrn4021
16. Guo, S., Wang, H., & Yin, Y. (2022). Microglia polarization From M1 to M2 in neurodegenerative Diseases. Frontiers in Aging Neuroscience, 14. doi:10.3389/fnagi.2022.815347
17. Wang, J., He, W., & Zhang, J. (2023). A richer and more diverse future for microglia phenotypes. Heliyon, 9(4). doi:10.1016/j.heliyon.2023.e14713
18. Hoshi, T., Toyama, T., Shinozaki, Y., Koizumi, S., Lee, J. Y., Naganuma, A., & Hwang, G. W. (2019). Evaluation of M1-microglial activation by neurotoxic metals using optimized organotypic cerebral slice cultures. The Journal of Toxicological Sciences, 44(7), 471–479. doi:10.2131/jts.44.471
19. Ding, Z. B., Song, L. J., Wang, Q., Kumar, G., Yan, Y. Q., & Ma, C. G. (2021). Astrocytes: A double-edged sword in neurodegenerative diseases. Neural Regeneration Research, 16(9), 1702–1710. doi:10.4103/1673-5374.306064
20. Kwon, H. S., & Koh, S. H. (2020). Neuroinflammation in neurodegenerative disorders: The roles of microglia and astrocytes. Translational Neurodegeneration, 9(1), 42. doi:10.1186/s40035-02000221-2
21. Adams, K. L., & Gallo, V. (2018). The diversity and disparity of the glial scar. Nature Neuroscience, 21(1), 9–15. doi:10.1038/s41593-017-0033-9
22. Sami, A., Selzer, M. E., & Li S. (2020). Advances in the signaling pathways downstream of glial-scar axon growth inhibitors. Frontiers in Cellular Neuroscience, 14. doi:10.3389/fncel.2020.00174
23. Mottahedin, A., Ardalan, M., Chumak, T., Riebe, I., Ek, J., & Mallard, C. (2017). Effect of neuroinflammation on synaptic organization and function in the developing brain: Implications for neurodevelopmental and neurodegenerative disorders. Frontiers in Cellular Neuroscience, 11. doi:10.3389/fncel.2017.00190
24. Yu, Z., Jiang, N., Su, W., & Zhuo, Y. (2021). Necroptosis: A novel pathway in neuroinflammation. Frontiers in Pharmacology, 12. doi:10.3389/ fphar.2021.701564
25. Wang, H., He, Y., Sun, Z., Ren, S., Liu, M., Wang, G., & Yang, J. (2022). Microglia in depression: An overview of microglia in the pathogenesis and treatment of depression. Journal of Neuroinflammation, 19(1). doi:10.1186/s12974-022-02492-0
26. Wang, J., Lai, S., Li, G., Zhou, T., Wang, B., Cao, F., Chen, T., Zhang, X., & Chen, Y. (2020). Microglial activation contributes to depressive-like behavior in dopamine D3 receptor knockout mice. Brain, Behavior, and Immunity, 83, 226–238. doi:10.1016/j.bbi.2019.10.016
27. Novakovic, M. M., Korshunov, K. S., Grant, R. A., Martin, M. E., Valencia, H. A., Budinger, G. R., Radulovic, J., & Prakriya, M. (2023). Astrocyte reactivity and inflammation-induced depression-like behaviors are regulated by Orai1 calcium channels. Nature Communications, 14(1). doi:10.1038/ s41467-023-40968-6
28. Bassett, B., Subramaniyam, S., Fan, Y., Varney, S., Pan, H., Carneiro, A. M. D., & Chung, C. Y. (2021). Minocycline alleviates depression-like symptoms by rescuing decrease in neurogenesis in dorsal hippocampus via blocking microglia activation/ phagocytosis. Brain, Behavior, and Immunity, 91, 519–530. doi:10.1016/j.bbi.2020.11.009
29. Soczynska, J. K., Kennedy, S. H., Alsuwaidan, M., Mansur, R. B., Li, M., McAndrews, M. P., Brietzke, E., Woldeyohannes, H. O., Taylor, V. H., & McIntyre, R. S. (2017). A pilot, open-label, 8-week study evaluating the efficacy, safety and tolerability of adjunctive minocycline for the treatment of bipolar I/II depression. Bipolar Disorders, 19(3), 198–213. doi:10.1111/bdi.12496
30. Nettis, M. A. (2021). Minocycline in major depressive disorder: And overview with considerations on treatment-resistance and comparisons with other psychiatric disorders. Brain, Behavior, & Immunity - Health, 17. doi:10.1016/j.bbih.2021.100335
31. Lu, L., Yang, L., Yue, J., Wang, X., Qi, J., Yang, F., Feng, B., & Liu, S. (2021). Scutellarin alleviates depression-like behaviors induced by LPS in mice partially through inhibition of astrocyte-mediated neuroinflammation. Neuroscience Letters, 765. doi:10.1016/j.neulet.2021.136284
32. Zhao, Q., Chen, X. Y., & Martin, C. (2016). Scutellaria baicalensis, the golden herb from the garden of Chinese medicinal plants. Science Bulletin, 61(18), 1391–1398. doi:10.1007/s11434016-1136-5
33. Wang, Q., Timberlake, M. A., Prall, K., & Dwivedi, Y. (2017). The recent progress in animal models of depression. Progress in Neuro-Psychopharmacology and Biological Psychiatry, 77, 99-109. doi:10.1016/j.pnpbp.2017.04.008
34. Xu, N., LaGrow, T. J., Anumba, N., Lee, A., Zhang, X., Yousefi, B., Bassil, Y., Clavijo, G. P., Khalilzad, S. V., Maltbie, E., Meyer-Baese, L., Nezafati, M., Pan, W. J., & Keilholz, S. (2022). Functional connectivity of the brain across rodents and humans. Frontiers in Neuroscience, 16. doi:10.3389/ fnins.2022.816331
35. Gencturk, S., & Unal, G. (2024). Rodent tests of depression and anxiety: Construct validity and translational relevance. Cognitive, Affective, & Behavioral Neuroscience, 24(2), 191–224. doi:10.3758/s13415-024-01171-2
36. Zhao, X., & Bhattacharyya, A. (2018). Human models are needed for studying human neurodevelopmental disorders. The American Journal of Human Genetics, 103(6), 829–857. doi:10.1016/j. ajhg.2018.10.009
37. Hollon, S. D., Cohen, Z. D., Singla, D. R., & Andrews, P. W. (2019). Recent developments in the treatment of depression. Behavior Therapy, 50(2), 257–269. doi:10.1016/j.beth.2019.01.002
38. Charkhandeh, M., Talib, M. A., & Hunt, C. J. (2016). The clinical effectiveness of cognitive behavior therapy and an alternative medicine approach in reducing symptoms of depression in adolescents. Psychiatry Research, 239, 325–330. doi:10.1016/j.psychres.2016.03.044
39. Ramic, E., Prasko, S., Gavran, L., & Spahic, E. (2020). Assessment of the antidepressant side effects occurrence in patients treated in primary care. Materia Socio-Medica, 32(2), 131–134. doi:10.5455/msm.2020.32.131-134
40. Alemi, F., Min, H., Yousefi, M., Becker, L. K., Hane, C. A., Nori, V. S., & Wojtusiak, J. (2021). Effectiveness of common antidepressants: a post market release study. EClinicalMedicine, 41. doi:10.1016/j. eclinm.2021.101171
41. Gartlehner, G., Gaynes B. N., Amick, H. R., Asher, G. N., Morgan, L. C., Coker-Schwimmer, E., Forneris, C., Boland E., Lux, L. J., Gaylord, S., Bann,C., Pierl, C. B., & Lohr, K. N. (2015). Comparative benefits and harms of antidepressant, psychological, complementary, and exercise treatments for major depression: An evidence report for a clinical practice guideline from the american college of physicians. Annals of Internal Medicine, 164(5), 331-341. doi:10.7326/M15-1813
42. Guo, B., Zhang, M., Hao, W., Wang, Y., Zhang, T., & Liu, C. (2023). Neuroinflammation mechanisms of neuromodulation therapies for anxiety and depression. Translational Psychiatry, 13(1). doi:10.1038/s41398-022-02297-y
43. Wang, Q., Zeng, L., Hong, W., Luo, M., Zhao, N., Hu, X., Shi, M., Qiu, J., Shen, Y., Teng, X., Min, H., & Liu, W. (2022). Inflammatory cytokines changed in patients with depression before and after repetitive transcranial magnetic stimulation treatment. Frontiers in Psychiatry, 13. doi:10.3389/ fpsyt.2022.925007
44. Kisely, S., Li, A., Warren, N., & Siskind, D. (2018). A systematic review and meta-analysis of deep brain stimulation for depression. Depression and Anxiety, 35(5), 468–480. doi:10.1002/da.22746
45. Amorim, B. O., Covolan, L., Ferreira, E., Brito, J. G., Nunes, D. P., de Morais, D. G., Nobrega, J. N., Rodrigues, A. M., deAlmeida, A. C. G., & Hamani, C. (2015). Deep brain stimulation induces antiapoptotic and anti-inflammatory effects in epileptic rats. Journal of Neuroinflammation, 12. doi:10.1186/s12974-015-0384-7
46. Chan, H. H., Wathen, C. A., Mathews, N. D., Hogue, O., Modic, J. P., Kundalia, R., Wyant, C., Park, H. J., Najm, I. M., Trapp, B. D., Machado, A. G., & Baker, K. B. (2018). Lateral cerebellar nucleus stimulation promotes motor recovery and suppresses neuroinflammation in a fluid percussion injury rodent model. Brain Stimulation, 11(6), 1356–1367. doi:10.1016/j.brs.2018.07.051
47. Dandekar, M. P., Saxena, A., Scaini, G., Shin, J. H., Migut, A., Giridharan, V. V., Zhou, Y., Barichello, T., Soares, J. C., Quevedo, J., & Fenoy, A. J. (2019). Medial forebrain bundle deep brain stimulation reverses anhedonic-like behavior in a chronic model of depression: Importance of BDNF and inflammatory cytokines. Molecular Neurobiology, 56(6), 4364–4380. doi:10.1007/s12035-018-1381-5
48. Fullana, N., Gasull-Camós, J., Tarrés-Gatius, M., Castañé, A., Bortolozzi, A., & Artigas, F. (2020). Astrocyte control of glutamatergic activity: Downstream effects on serotonergic function and emotional behavior. Neuropharmacology, 166. doi:10.1016/j.neuropharm.2019.107914
1. Hoffman, K. M., Trawalter, S., Axt, J. R., & Oliver, M. N. (2016). Racial bias in pain assessment and treatment recommendations, and false beliefs about biological differences between blacks and whites. Proceedings of the National Academy of Sciences, 113(16), 4296-4301. doi:10.1073/ pnas.1516047113
2. Rastegar, P., Cai, L., & Langhinrichsen-Rohling, J. (2025). Racial discrimination as a traumatic bedrock of healthcare avoidance: A pathway through healthcare institutional betrayal and mistrust. Healthcare, 13(5), 486. doi:10.3390/ healthcare13050486
3. Booker, S. Q., Merriwether, E. N., Powell-Roach, K., & Jackson, S. (2024). From stepping stones to scaling mountains: Overcoming racialized disparities in pain management. Pain Management, 14(1), 5-12. doi:10.2217/pmt-2023-0098
4. Egan, K. (2024). The roots and clinical effects of racial bias in medicine. University of Michigan Undergraduate Research Journal, 17(1), 26-36. doi:10.3998/umurj.5504
5. Bailey, Z. D., Feldman, J. M., & Bassett, M. T. (2021). How structural racism works – Racist policies as a root cause of U.S. racial health inequities. The New England Journal of Medicine, 384(8), 768-773. doi:10.1056/NEJMms2025396
6. Trawalter, S., & Hoffman, K. M. (2015). Got pain? Racial bias in perceptions of pain. Social and Personality Psychology Compass, 9(3), 146-157. doi:10.1111/spc3.12161
7. Vernon, L. F. (2019). J. Marion Sims, MD: Why he and his accomplishments need to continue to be recognized a commentary and historical review. Journal of the National Medical Association, 111(4), 436-446. doi:10.1016/j.jnma.2019.02.002
8. Wailoo, K. (2018). Historical aspects of race and medicine: The case of J. Marion Sims. Journal of the American Medical Association, 320(15), 15291530. doi:10.1001/jama.2018.11944
9. Deb, B., & Rodman, A. (2024). Racial differences in pain assessment and false beliefs about race in AI models. Journal of the American Medical Association Network Open, 7(10). doi:10.1001/jamanetworkopen.2024.37977
10. Hall, O. T., Jordan, A., Teater, J., Dixon-Shambley, K., McKiever, M. E., Baek, M., Garcia, S., Rood, K. M., & Fielin, D. A. (2022). Experiences of racial discrimination in the medical setting and associations with medical mistrust and expectation of care among black patients seeking addiction treatment. Journal of Substance Abuse Treatment, 113(1). doi:10.1016/j.jsat.2021.108551
11. Satcher, L. A. (2022). (Un)Just deserts: Examining resource deserts and the continued significance of racism on health in the urban South. Sociology of Race and Ethnicity, 8(4), 483-502. doi:10.1177/23326492221112424
12. Stepanikova, I., & Oates, G. R. (2017). Perceived discrimination and privilege in health care: The role of socioeconomic status and race. American Journal of Preventive Medicine, 52(1), 86-94. doi:10.1016/j.amepre.2016.09.024
13. Macias-Konstantopoulos, W. L., Collins, K. A., Diaz, R., Duber, H. C., Edwards, C. D., Hsu, A. P., Ranney, M. L., Riviello, R. J., Wettstein, Z. S., & Sachs, C.J. (2023). Race, healthcare, and health disparities: A critical review and recommendation for advancing health equity. Western Journal of Emergency Medicine: Integrating Emergency Care with Population Health, 24(5), 906-918. doi:10.5811/westjem.58408
14. Braveman, P. A., Arkin, E., Proctor, D., Kauh, T., & Holm, N. (2022). Systemic and structural racism: Definitions, examples, health damages, and approaches to dismantling. Health Affairs, 41(2), 171-178. doi:10.1377/hlthaff.2021.01394
15. Ghoshal, M., Shapiro, H., Todd, K., & Schatman, M. E. (2020). Chronic noncancer pain management and systemic racism: Time to move toward equal care standards. Journal of Pain Research, 13(1), 2825-2836. doi:10.2147/JPR.S287314
16. Walker Taylor, J. L., Campbell, C. M., Thorpe Jr., R. J., Whitfield, K. E., Nkimbeng, M., & Szanton, S. L. (2018). Pain, racial discrimination, and depressive symptoms among African American women. Pain Management Nursing, 19(1), 79-87. doi:10.1016/j. pmn.2017.11.008
17. Bazargan, M., Cobb, S., Assari, S., & Bazargan-Hejazi, S. (2022). Physical and mental health quality of life among underserved African American and Latino older adults. Ethnicity & Health, 28(2), 217-233. doi:10.1080/13557858.2022.2027886
18. Guadamuz, J. S., Wilder, J. R., Mouslim, M. C., Zenk, S. N., Alexander, G. C., & Qato, D. M. (2021). Fewer pharmacies in Black and Hispanic/Latino neighborhoods compared with White or diverse neighborhoods, 2007-15. Health Affairs, 40(5), 802-811. doi:10.1377/hlthaff.2020.01699
19. Brînzac, M. G., Kuhlmann, E., Dussault, G., Ungureanu, M. I., Cherecheș, R. M., & Baba, C. O. (2023). Defining medical deserts–An international consensus-building exercise. European Journal of Public Health, 33(5), 785-788. doi:10.1093/eurpub/ckad107
20. Chisholm-Burns, M. A., Spivey, C. A., Gatwood, J., Wiss, A., Hohmeier, K., & Erickson, S. R. (2017). Evaluation of racial and socioeconomic disparities in medication pricing and pharmacy access and services. American Journal of Health-System Pharmacy, 74(19), 653-668. doi:10.2146/ ajhp150872
21. Ganguli, I., Mackwood, M. B., Yang, C. W., Crawford, M., Mulligan, K. L., O’Malley, A. J., Fisher, E. S., & Morden, N. E. (2023). Racial differences in low value care among older adult Medicare patients in US health systems: Retrospective cohort study. British Medical Journal, 383(1). doi:10.1136/bmj-2023-074908
22. Yearby, R., Clark, B., & Figueroa, J. F. (2022). Structural racism in historical and modern US health care policy. Health Affairs, 41(2), 187-194. doi:10.1377/hlthaff.2021.01466
23. Alba, C., Zheng, Z., & Wadhera, R. K. (2024). Changes in health care access and preventive health screenings by race and ethnicity. Journal of the American Medical Association Health Forum, 5(2). doi:10.1001/jamahealthforum.2023.5058
24. Powell, M., Richmond, J., Mohottige, D., Yen, I., Joslyn, A., & Corbie-Smith, G. (2019). Medical mistrust, racism, and delays in preventive health screening among African-American men. Behavioral Medicine, 45(2), 102-117. doi:10.1080/089642 89.2019.1585327
25. Siegel, R. L., Kratzer, T. B., Giaquinto, A. N., & Sung, H. (2025). Cancer statistics, 2025. CA: A Cancer Journal for Clinicians, 75(1), 10-45. doi:10.3322/caac.21871
26. Kurumety, S. K., Howshar, J. T., & Loving, V. A. (2023). Breast cancer screening and outcomes disparities persist for Native American women. Journal of Breast Imaging, 5(1), 3-10. doi:10.1093/ jbi/wbac080
27. Roubidoux, M. A., Kaur, J. S., & Rhoades, D. A. (2022). Health disparities in cancer among American Indians and Alaska natives. Academic Radiology, 29(7), 1013-1021. doi:10.1016/j. acra.2021.10.011
28. Ginsburg, O., Yip, C., Brooks, A., Cabanes, A., Caleffi, M., Dunstan Yataco, J. A., Gyawali, B., McCormack, V., Murillo, R., Pace, L. E., Paskett, E. D., Romanoff, A., Unger-Saldaña, K, Vanderpuye, V., Wu, T., Yuma, S., Dvaladze, A., Duggan, C., & Anderson, B. O. (2020). Breast cancer early detection: a phased approach to implementation. Cancer, 126(S10), 2379-2393. doi:10.1002/ cncr.32887
29. Crosby, D., Bhatia, S., Brindle, K. M., Coussens, L. M., Dive, C., Emberton, M., Esener, S., Fitzgerald, R. C., Gambhir, S. S., Kuhn, P., Rebbeck, T. R., & Balasubramanian, S. (2022). Early detection of cancer. Science, 375(6586). doi:10.1126/science. aay9040
30. Cosgrove, G. P., Bianchi, P., Danese, S., & Lederer, D. J. (2018). Barriers to timely diagnosis of interstitial lung disease in the real world: The INTENSITY survey. BMC Pulmonary Medicine, 18(9). doi:10.1186/s12890-017-0560-x
31. Star, J., Bandi, P., Nargis, N., Islami, F., Yabroff, K. R., Minihan, A. K., Smith, R. A., & Jemal, A. (2023). Updated review of major cancer risk factors and screening test use in the United States, with a focus on changes during the COVID-19 pandemic. Cancer Epidemiology, Biomarkers & Prevention, 32(7), 879-888. doi:10.1158/1055-9965. EPI-23-0114
32. Doan, L. V., Yoon, J., Chun, J., Perez, R., & Wang, J. (2023). Pain associated with breast cancer: Etiologies and therapies. Frontiers in Pain Research, 4(1). doi:10.3389/fpain.2023.1182488
33. Kamgar, M., Assad, H., Hastert, T. A., McLaughlin, E., Reding, K., Paskett, E. D., Bea, J. W., Shadyab, A. H., Neuhouser, M. L., Nassir, R., Crane, T. E., Sreeram, K., Greenwald, M. K., & Simon, M. S. (2020). Peripheral neuropathy after breast cancer: An analysis of data from the Women’s Health Initiative Life and Longevity After Cancer cohort. Journal of Clinical Oncology, 38(15). doi:10.1200/ JCO.2020.38.15_suppl.e24093
34. Colosia, A., Njue, A., Bajwa, Z., Dragon, E., Robinson, R. L. , Sheffield, K. M., Thakkar, S., & Richiemer, S. H. (2022). The burden of metastatic cancer-induced bone pain: A narrative review. Journal of Pain Research, 15, 3399-3412. doi:10.2147/JPR.S371337
35. Jin, L., Han, B., Siegel, E., Cui, Y., Giuliano, A., & Cui, X. (2018). Breast cancer lung metastasis: Molecular biology and therapeutic implications. Cancer Biology & Therapy, 19(10), 858-868. doi:10. 1080/15384047.2018.1456599
36. Danforth, D. (2021). The role of chronic inflammation in the development of breast cancer. Cancers, 13(15), 3918. doi:10.3390/cancers13153918
37. Nishigami, T., Manfuku, M., & Lahousse, A. (2023). Central sensitization in cancer survivors and its clinical implications: State of the art. Journal of Clinical Medicine, 12(14). doi:10.3390/jcm12144606
38. Schwab, L., & Visovsky, C. (2023). Psychological distress and quality of life in breast cancer survivors with taxane-induced peripheral neuropathy: A scoping review. Frontiers in Oncology, 12(1). doi:10.3389/fonc.2022.1005083
39. Mestdagh, F., Steyaert, A., & Lavand’homme, P. (2023). Cancer pain management: A narrative review of current concepts, strategies, and techniques. Current Oncology, 30(7), 6838-6858. doi:10.3390/curroncol30070500
40. Overstreet, D. S., Pester, B. D., Wilson, J. M., Flowers, K. M., Kline, N. K., & Meints, S. M. (2022). The experience of BIPOC living with chronic pain in the USA: Biopsychosocial factors that underlie racial disparities in pain outcomes, comorbidities, inequities, and barriers to treatment. Current Pain and Headache Reports, 27(1), 1-10. doi:10.1007/s11916-022-01098-8
41. Pham, T. V., Doorley, J., Kenney, M., Joo, J. H., Shallcross, A. J., Kincade, M., Jackson, J., & Vranceanu, A. M. (2023). Addressing chronic pain disparities between Black and White people: A narrative review of socio-ecological determinants. Pain Management, 13(8), 473–496. doi:10.2217/pmt-2023-0032
42. Chin, M. H., Afsar-Manesh, N., Bierman, A. S., Chang, C., Colón-Rodríguez, C. J., Dullabh, P., Duran, D. G., Fair, M., Hernandez-Boussard, T., Hightower, M., Jain, A., Jordan, W. B., Konya, S., Moore, R. H., Moore, T. T., Rodriguez, R., Shaheen, G., Snyder, L. P., Srinivasan, M., Umscheid, C. A., & Ohno-Machado, L. (2023). Guiding principles to address the impact of algorithm bias on racial and ethnic disparities in health and health care. Journal of the American Medical Association Network Open, 6(12). doi:10.1001/jamanetworkopen.2023.45050
43. Canedo, J. R., Miller, S. T., Myers, H. F., & Sanderson, M. (2019). Racial and ethnic differences in knowledge and attitudes about genetic testing in the US: Systematic review. Journal of Genetic Counseling, 28(3), 587-601. doi:10.1002/jgc4.1078
44. Obermeyer, Z., Powers, B., Vogeli, C., & Mullainathan, S. (2019). Dissecting racial bias in an algorithm used to manage the health of populations. Science, 366(6464), 447-453. doi:10.1126/science. aax2342
45. Varnosfaderani, S. M., & Forouzanfar, M. (2024). The role of AI in hospitals and clinics: Transforming healthcare in the 21st century. Bioengineering, 11(4), 337. doi:10.3390/bioengineering11040337
46. El-Azab, S., & Nong, P. (2023). Clinical algorithms, racism, and “fairness” in healthcare: A case of bounded justice. Big Data & Society, 10(2). doi:10.1177/20539517231213820
47. Liu, P., Sawhney, S., Heide-Jørgensen, U., Quinn, R. R., Jensen, S. K., Mclean, A., Christiansen, C. F., Gerds, T. A., & Ravani, P. (2024). Predicting the risks of kidney failure and death in adults with moderate to severe chronic kidney disease: Multinational, longitudinal, population based, cohort study. British Medical Journal, 385(1). doi:10.1136/ bmj-2023-078063
48. Huang, T., Shu, Y. & Cai, Y. D. (2015). Genetic differences among ethnic groups. BMC Genomics, 16. doi:10.1186/s12864-015-2328-0
49. Letzen, J. E., Mathur, V. A., Janevic, M. R., Burton, M. D., Hood, A. M., Morais, C. A., Booker, S. Q., Campbell, C. M., Aroke, E. N., Goodin, B. R., Campbell, L. C., & Merriwether, E. N. (2022). Confronting racism in all forms of pain research: Reframing study designs. The Journal of Pain, 23(6), 893–912. doi:10.1016/j.jpain.2022.01.010
50. Timbrell, N. E. (2024). The role and limitations of the reference interval within clinical chemistry and its reliability for disease detection. British Journal of Biomedical Science, 81(1). doi:10.3389/ bjbs.2024.12339
51. Jain, A., Brooks, J. R., Alford, C. C., Chang, C. S., Mueller, N. M., Umscheid, C. A., & Bierman, A. S. (2023). Awareness of racial and ethnic bias and potential solutions to address bias with use of health care algorithms. Journal of American Medical Association Health Forum, 4(6). doi:10.1001/jamahealthforum.2023.1197
52. Rappoport, N., Paik, H., Oskotsky, B., Tor, R., Ziv, E., Zaitlen, N., & Butte, A. J. (2018). Comparing ethnicity-specific reference intervals for clinical laboratory tests from EHR data. The Journal of Applied Laboratory Medicine, 3(3), 366–377. doi:10.1373/jalm.2018.026492
53. Wong, N. D., Budoff, M. J., Ferdinand, K., Graham, I. M., Michos, E. D., Reddy, T., Shapiro, M. D., & Toth, P. P. (2022). Atherosclerotic cardiovascular disease risk assessment: An American Society for Preventive Cardiology clinical practice statement. American Journal of Preventive Cardiology, 10(1). doi:10.1016/j.ajpc.2022.100335
54. Anderson, T. S., Wilson, L. M., & Sussman, J. B. (2024). Atherosclerotic cardiovascular disease risk estimates using the predicting risk of cardiovascular disease events equations. Journal of American Medical Association Internal Medicine, 184(8), 963–970. doi:10.1001/jamainternmed.2024.1302
55. Bansal, M., Ranjan, S.,& Kasliwal, R. R. (2020). Cardiovascular risk calculations and their applicability to South Asians. Current Diabetes Reviews, 17(9). doi:10.2174/157339981699920100120 4020
56. Palaniappan, L., Garg, A., Enas, E., Lewis, H., Bari, S., Gulati, M., Flores, C., Mathur, A., Molina, C., Narula, J., Rahman, S., Leng, J., & Gany, F. (2018). South Asian cardiovascular disease & cancer risk: Genetics & pathophysiology. Journal of Community Health, 43(1), 1100–1114. doi:10.1007/s10900018-0527-8
57. Shah, K. S., Patel, J., Al Rifai, M., Agarwala, A., Bhatt, A. B., Levitzky, Y. S., & Palaniappan, L. (2022). Cardiovascular risk management in the South Asian patient: A review. Health Sciences Review, 4(1). doi:10.1016/j.hsr.2022.100045
58. Patel, A. P., Wang, M., Kartoun, U., Ng, K., & Khera, A. V. (2021). Quantifying and understanding the higher risk of atherosclerotic cardiovascular disease among South Asian individuals. Circulation, 144(6), 410–422. doi:10.1161/CIRCULATIONAHA.120.052430
59. Kalra, D., Vijayaraghavan, K., Sikand, G., Desai, N. R., Joshi, P. H., Mehta, A., Karmally, W., Vani, A., Sitafalwalla, S. J., Puri, R., Duell, P. B., & Brown, A. (2021). Prevention of atherosclerotic cardiovascular disease in South Asians in the US: A clinical perspective from the National Lipid Association. Journal of Clinical Lipidology, 15(3), 402–422. doi:10.1016/j.jacl.2021.03.007
60. Nanayakkara, G. L., Rai, T., Kirincic, L., Lightfoot, R., Senaratne, J. M., & Senaratne, M. (2021). Differences in clinical measures and outcomes in South Asians vs Caucasians attending cardiac rehabilitation. CJC Open, 3(8), 1019–1024. doi:10.1016/j.cjco.2021.03.014
61. Makover, M. E., Shapiro, M. D., & Toth, P. P. (2022). There is urgent need to treat atherosclerotic cardiovascular disease risk earlier, more intensively, and with greater precision: A review of current practice and recommendations for improved effectiveness. American Journal of Preventive Cardiology, 12(1). doi:10.1016/j.ajpc.2022.100371
62. Jurgens, C. Y., Lee, C. S., Aycock, D. M., Masterson Creber, R., Denfeld, Q. E., DeVon, H. A., Evers, L. R., Jung, M., Pucciarelli, G., Streur, M. M., & Konstam, M. A. (2022). State of the science: The relevance of symptoms in cardiovascular disease and research: a scientific statement from the American Heart Association. Circulation, 146(12), e173–e184. doi:10.1161/CIR.0000000000001089
63. Jumreornvong, O., Perez, A. M., Malave, B., Mozawalla, F., Kia, A., & Nwaneshiudu, C.A. (2025). Biases in artificial intelligence application in pain medicine. Journal of Pain Research, 18, 10211033. doi:10.2147/JPR.S495934
64. Bayne, J., Garry, J., & Albert, M.A. (2023). Brief review: Racial and ethnic disparities in cardiovascular care with a focus on congenital heart disease and precision medicine. Current Atherosclerosis Reports, 25(1), 189–195. doi:10.1007/ s11883-023-01093-3
65. Agarwal, A. K., Gonzales, R. E., Sagan, C., Nijim, S., Asch, D. A., Merchant, R. M., & South, E. C. (2024). Perspectives of Black patients on racism within emergency care. Journal of American Medical Association Health Forum, 5(3). doi:10.1001/jamahealthforum.2024.0046
66. Goldfarb, S. S., Graves, K., Geletko, K., Hansen, M. D., Kinsell, H., & Harman, J. (2023). Racial and ethnic differences in emergency department wait times for patients with substance use disorder. The Journal of Emergency Medicine, 64(4), 481–487. doi:10.1016/j.jemermed.2023.02.015
67. Zhang, X., Carabello, M., Hill, T., Bell, S. A., Stephenson, R., & Mahajan, P. (2020). Trends of racial/ethnic differences in emergency department care outcomes among adults in the United States from 2005 to 2016. Frontiers in Medicine, 7(300). doi:10.3389/fmed.2020.00300
68. Qiao, W. P., Powell, E. S., Witte, M. P., & Zelder, M. R. (2016). Relationship between racial disparities in ED wait times and illness severity. The American Journal of Emergency Medicine, 34(1), 10–15. doi:10.1016/j.ajem.2015.08.052
69. Ikeme, S., Kottenmeier, E., Uzochukwu, G., & Brinjikji, W. (2022). Evidence-based disparities in stroke care metrics and outcomes in the United States: A systematic review. Stroke, 53(3), 670–679. doi:10.1161/STROKEAHA.121.036263
70. Chang, T., Nuppnau, M., He, Y., Kocher, K. E., Valley, T. S., Sjoding, M. W., & Wiens, J. (2024). Racial differences in laboratory testing as a potential mechanism for bias in AI: A matched cohort analysis in emergency department visits. PLOS Global Public Health, 4(10). doi:10.1371/journal. pgph.0003555
71. Gopal, D. P., Chetty, U., O’Donnell, P., Gajria, C., & Blackadder-Weinstein, J. (2021). Implicit bias in healthcare: Clinical practice, research and decision making. Future Healthcare Journal, 8(1), 40–48. doi:10.7861/fhj.2020-0233
72. Ceccarelli, I., Bagnis, A., Ottaviani, C., Thayer, J. F., & Mattarozzi, K. (2024). Racial biases, facial trustworthiness, and resting heart rate variability: Unravelling complexities in pain recognition. Cognitive Research: Principles and Implications, 9(1). doi:10.1186/s41235-024-00588-0
73. Boley, S., Sidebottom, A., Vacquier, M., Watson, D., Olsen, J., Echols, K., & Friedman, S. (2022). Investigating racial disparities within an emergency department rapid-triage system. The American Journal of Emergency Medicine, 60(1), 65–72. doi:10.1016/j.ajem.2022.07.030
74. Agarwal, A. K., Gonzales, R. E., Sagan, C., Nijim, S., Asch, D. A., Merchant, R. M., & South, E. C. (2024). Perspectives of Black patients on racism within emergency care. Journal of American Medical Association Health Forum, 5(3). doi:10.1001/jamahealthforum.2024.0046
75. Nguyen, L. H., Dawson, J. E., Brooks, M., Khan, J. S., & Telusca, N. (2023). Disparities in pain management. Anesthesiology Clinics, 41(2), 471–488. doi:10.1016/j.anclin.2023.03.008
76. Hausmann, L. R. M., Jones, A. L., McInnes, S. E., & Zickmund, S. L. (2020). Identifying healthcare experiences associated with perceptions of racial/ ethnic discrimination among veterans with pain: A cross-sectional mixed methods survey. PLOS one, 15(9). doi:10.1371/journal.pone.0237650
77. Strand, N. H., Mariano, E. R., Goree, J. H., Narouze, S., Doshi, T. L., Freeman, J. A., & Pearson, A. C. S. (2021). Racism in pain medicine: We can and should do more. Mayo Clinic Proceedings, 96(6), 1394–1400. doi:10.1016/j.mayocp.2021.02.030
78. Morden, N. E., Chyn, D., Wood, A., & Meara, E. (2021). Racial inequality in prescription opioid receipt—Role of individual health systems. New England Journal of Medicine, 385(4), 342–351. doi:10.1056/NEJMsa2034159
79. Drake, J., Charles, C., Bourgeois, J. W., Daniel, E. S., & Kwende, M. (2020). Exploring the impact of the opioid epidemic in Black and Hispanic communities in the United States. Drug Science, Policy and Law, 6(1). doi:10.1177/2050324520940428
80. Gondré-Lewis, M. C., Abijo, T., & Gondré-Lewis, T. A. (2023). The opioid epidemic: A crisis disproportionately impacting Black Americans and urban communities. Journal of Racial and Ethnic Health Disparities, 10(4), 2039–2053. doi:10.1007/ s40615-022-01384-6
81. Judd, D., King, C. R., & Glake, C. (2023). The opioid epidemic: A review of the contributing factors, negative consequences, and best practices. Cureus, 15(7). doi:10.7759/cureus.41621
82. Jannetto, P. J. (2021). The North American opioid epidemic. Therapeutic Drug Monitoring, 43(1), 1-5. doi:10.1097/FTD.0000000000000817
83. Santoro, T. N., & Santoro, J. D. (2018). Racial bias in the US opioid epidemic: A review of the history of systemic bias and implications for care. Cureus, 10(12). doi:10.7759/cureus.3733
84. Armenian, P., Vo, K., Barr-Walker, J., Lynch (2018). Fentanyl, fentanyl analogs and novel synthetic opioids: A comprehensive review. Neuropharmacology, 134(A), 121-132. doi:10.1016/j.neuropharm.2017.10.016
85. Ghonasgi, R., Paschke, M. E., Winograd, R. P., Wright, C., Selph, E., & Banks, D. E. (2024). The intersection of substance use stigma and anti-Black racial stigma: A scoping review. International Journal of Drug Policy, 133(1). doi:10.1016/j. drugpo.2024.104612
86. Morden, N. E.; Chyn, D.; Wood, A.; Meara, E. Racial Inequality in Prescription Opioid Receipt — Role of Individual Health Systems. New England Journal of Medicine 2021, 385 (4), 342–351. DOI:10.1056/nejmsa2034159.
87. Schroeder, A. R., Dehghan, M., Newman, T. B., Bentley, J. P., Park, K. T. (2019). Association of opioid prescriptions from Dental clinicians for US adolescents and young adults with subsequent opioid use and abuse. Journal of American Medical Association Internal Medicine, 179(2), 145–152. doi:10.1001/jamainternmed.2018.5419
88. Compton, W. M., Jones, C. M., & Baldwin, G. T. (2016). Relationship between nonmedical prescription-opioid use and heroin use. New England Journal of Medicine, 374(2), 154–163. doi:10.1056/NEJMra1508490
89. Pyra, M., Taylor, B., Flanagan, E., Hotton, A., Johnson, O., Lamuda, P., Schneider, J., & Pollack, H. A. (2022). Support for evidence-informed opioid policies and interventions: The role of racial attitudes, political affiliation, and opioid stigma. Preventive Medicine, 158(1). doi:10.1016/j. ypmed.2022.107034
90. James, K., & Jordan, A. (2018). The opioid crisis in Black communities. Journal of Law, Medicine & Ethics, 46(2), 404–421. doi:10.1177/1073110518782949
91. Bazargan, M., Cobb, S., & Assari, S. (2021). Discrimination and medical mistrust in a racially and ethnically diverse sample of California adults. The Annals of Family Medicine, 19(1), 4. doi:10.1370/afm.2632
92. Goyal, M. K., Kuppermann, N., Cleary, S. D., Teach, S. J., & Chamberlain, J. M. (2015). Racial disparities in pain management of children with appendicitis in emergency departments. Journal of American Medical Association Pediatrics, 169(11), 996–1002. doi:10.1001/jamapediatrics.2015.1915
93. Badreldin, N., Grobman, W. A., & Yee, L. M. (2019). Racial disparities in postpartum pain management. Obstetrics & Gynecology, 134(6), 1147-1153. doi:10.1097/AOG.0000000000003561
94. Johnson, J. D., Asiodu, I. V., McKenzie, C. P., Tucker, C., Tully, K. P., Bryant, K., Verbiest, S., & Stuebe, A. M. (2019). Racial and ethnic inequities in postpartum pain evaluation and management. Obstetrics & Gynecology, 134(6), 1155-1162. doi:10.1097/AOG.0000000000003505
95. Bornstein, E., Eliner, Y., Chervenak, F. A., & Grünebaum, A. (2020). Racial disparity in pregnancy risks and complications in the US: Temporal changes during 2007–2018. Journal of Clinical Medicine, 9(5). doi:10.3390/jcm9051414
96. Calcutt, N. A. (2020). Diabetic neuropathy and neuropathic pain: A (con)fusion of pathogenic mechanisms? PAIN, 161(1). doi:10.1097/j. pain.0000000000001922
97. Lipman, T. H., Smith, J. A., Patil, O.,Willi, S. M., & Hawkes, C. P. (2020). Racial disparities in treatment and outcomes of children with type 1 diabetes. Pediatric Diabetes, 22(2), 241–248. doi:10.1111/pedi.13139
98. Meints, S. M., Cortes, A., Morais, C. A., & Edwards, R. R. (2019). Racial and ethnic differences in the experience and treatment of noncancer pain. Pain Management, 9(3), 317–334. doi:10.2217/pmt2018-0030
99. Thompson, T., Stathi, S., Shin, J. I., Carvalho, A., Solmi, M., & Liang, C. S. (2024). Racial and ethnic disparities in the prescribing of pain medication in US primary care settings, 1999–2019: Where are we now? Journal of General Internal Medicine, 39(9), 1597–1605. doi:10.1007/s11606-02408638-5
100. Gonzalez, C. M., Ark, T. K., Fisher, M. R., Marantz, P. R., Burgess, D. J., Milan, F., Samuel, M. T., Lypson, M. L., Rodriguez, C. J., & Kalet, A. L. (2024). Racial implicit bias and communication among physicians in a simulated environment. Journal of American Medical Association Network Open, 7(3). doi:10.1001/jamanetworkopen.2024.2181
101. Mhadhbi, H., MacMillan, A., Draper-Rodi, J., Ménard, M., & Sposato, N. S. (2025). Advancing equitable osteopathic practice: integrating person-centredness & addressing racial biases through the lens of critical theory. International Journal of Osteopathic Medicine, 55(1). doi:10.1016/j.ijosm.2025.100752
102. Jayathilake, N. J., Phan, T. T., Kim, J., Lee, K. P., & Park, J. M. (2025). Modulating neuroplasticity for chronic pain relief: Noninvasive neuromodulation as a promising approach. Experimental & Molecular Medicine, 57(3), 501–514. doi:10.1038/ s12276-025-01409-0
103. Dodds, K. N., Beckett, E. A. H., Evans, S. F., Grace, P. M., Watkins, L. R., & Hutchinson, M. R. (2016). Glial contributions to visceral pain: implications for disease etiology and the female predominance of persistent pain. Translational Psychiatry, 6(9). doi:10.1038/tp.2016.168
104. Dinakar, P., & Stillman, A. M. (2016). Pathogenesis of pain. Seminars in Pediatric Neurology, 23(3), 201–208. doi:10.1016/j.spen.2016.10.003
105. Volcheck, M. M., Graham, S. M., Fleming, K. C., Mohabbat, A. B., & Luedtke, C. A. (2023). Central sensitization, chronic pain, and other symptoms: Better understanding, better management. Cleveland Clinic Journal of Medicine, 90(4), 245254. doi:10.3949/ccjm.90a.22019
106. Van Griensven, H., Schmid, A., Trendafilova, T., & Low, M. (2020). Central sensitization in musculoskeletal pain: Lost in translation? Journal of Orthopaedic & Sports Physical Therapy, 50(11), 592-596. doi:10.2519/jospt.2020.0610
107. Armstrong, M., Castellanos, J., & Christie, D. (2024). Chronic pain as an emergent property of a complex system and the potential roles of psychedelic therapies. Frontiers in Pain Research, 5(1). doi:10.3389/fpain.2024.1346053
108. Borsook, D., Youssef, A. M., Simons, L., Elman, I., & Eccleston, C. (2018). When pain gets stuck: The evolution of pain chronification and treatment resistance. PAIN, 159(12), 2421-2436. doi:10.1097/j.pain.0000000000001401
109. Hobson, J. M., Moody, M. D., Sorge, R. E., & Goodin, B. R. (2022). The neurobiology of social stress resulting from racism: Implications for pain disparities among racialized minorities. Neurobiology of Pain, 12(1). doi:10.1016/j.ynpai.2022.100101
110. Hood, A. M., Morais, C. A., Fields, L. N., Merriwether, E. N., Brooks, A. K., Clark, J. F., McGill, L. S., Janevic, M. R., Letzen, J. E., & Campbell, L. C. (2023). Racism exposure and trauma accumulation perpetuate pain inequities–Advocating for change (RESTORATIVE): A conceptual model. American Psychologist, 78(2), 143-159. doi:10.1037/amp0001042
111. Paradies, Y., Ben, J., Denson, N., Elias, A., Priest, N., Pieterse, A., Gupta, A., Kelaher, M., & Gee, G. (2015). Racism as a determinant of health: A systematic review and meta-analysis. PLOS One, 10(9). doi:10.1371/journal.pone.0138511
112. Duenas, M., Ojeda, B., Salazar, A., Mico, J. A., & Failde, I. (2016). A review of chronic pain impact on patients, their social environment and the health care system. Journal of Pain Research, 2016(9), 457-467. doi:10.2147/JPR.S105892
113. Oakley, L. P., Harvey, S. M., & López-Cevallos, D. F. (2018). Racial and ethnic discrimination, medical mistrust, and satisfaction with birth control services among young adult Latinas. Women’s Health Issues, 28(4), 313–320. doi:10.1016/j. whi.2018.03.007
114. Alsan, M., Wanamaker, M., & Hardeman, R.R. (2020). The Tuskegee study of untreated syphilis: A case study in peripheral trauma with implications for health professionals. Journal of General Internal Medicine, 35(1), 322–325. doi:10.1007/ s11606-019-05309-8
115. Paul, C., & Brookes, B. (2015). The rationalization of unethical research: revisionist accounts of the Tuskegee Syphilis Study and the New Zealand “unfortunate experiment.” American Journal of Public Health, 105(10). doi:10.2105/ AJPH.2015.302720
116. Tobin, M. J. (2022). Fiftieth anniversary of uncovering the Tuskegee Syphilis Study: The story and timeless lessons. American Journal of Respiratory and Critical Care Medicine, 205(10), 1145–1158. doi:10.1164/rccm.202201-0136SO
117. Stamm L. V. (2016). Syphilis: Re-emergence of an old foe. Microbial Cell, 3(9), 363–370. doi:10.15698/mic2016.09.523
118. Reverby, S. (2022). The Tuskegee Syphilis Study. Oxford Research Encyclopedia of American History. doi:10.1093/acrefore/9780199329175.013.1032
119. Alsan, M., & Wanamaker, M. (2018). Tuskegee and the health of Black men. The Quarterly Journal of Economics, 133(1), 407–455. doi:10.1093/qje/ qjx029
120. O’Brien, E., & Rich, M. (2022). Obstetric violence in historical perspective. The Lancet, 399(10342), 2183–2185. doi:10.1016/S01406736(22)01022-4
121. Novak, N. L., Lira, N., O’Connor, K. E., Harlow, S. D., Kardia, S. L. R., & Stern, A. M. (2018). Disproportionate sterilization of Latinos under California’s eugenic sterilization program, 1920–1945. American Journal of Public Health, 108(5), 611–613. doi:10.2105/AJPH.2018.304369
122. Schickler, R., Whittum, M., Fanarjian, N., Rapkin, R., & Nguyen, B. T. (2021). The history of female surgical sterilization: A social and ethics perspective. Journal of Gynecologic Surgery, 37(6), 465–469. doi:10.1089/gyn.2021.0102
123. Leason, J. (2021). Forced and coerced sterilization of Indigenous women. Canadian Family Physician, 67(7), 525. doi:10.46747/cfp.6707525
124. Sutton, M. Y., Anachebe, N. F., Lee, R., & Skanes, H. (2021). Racial and ethnic disparities in reproductive health services and outcomes, 2020. Obstetrics & Gynecology, 137(2), 225-233. doi:10.1097/AOG.0000000000004224
125. Averbach, S., Ha, D., Meadows, A., Brubaker, L., & Gyamfi-Bannerman, C. (2023). Failure to progress: Structural racism in women’s healthcare. eClinicalMedicine, 57(1), 101861. doi:10.1016/j. eclinm.2023.101861
126. Miller, F., & Miller, P. (2021). Transgenerational trauma and trust restoration. American Medical Association Journal of Ethics, 23(6), 480-486. doi:10.1001/amajethics.2021.480
127. Garvick, S. J., Banz, J., Chin, M., Fesler, K., Olson, A. M., Wolff, E., & Gregory, T. (2023). Racial disparities in pain management: Historical maleficence and solutions for equity. JAAPA, 36(11), 37-41. doi:10.1097/01.JAA.0000979472.53675.b6
128. Keeys, M., Baca, J., & Maybank, A. (2021). Race, racism, and the policy of 21st century medicine. Yale Journal of Biology and Medicine, 94(1), 153-157. PMID:33795992
FEATURED THE FUTURE OF THOUGHT: NEURALINK AND BRAIN COMPUTER INTERFACES
1. Vilela, M. & Hochberg, L. R. (2020). Applications of brain-computer interfaces to the control of robotic and prosthetic arms. Handbook of Clinical Neurology, 186, 87-99. doi:10.1016/B978-0444-63934-9.00008-1.
2. Mridha, M. F., Das, S. C., Kabir, M. M., Lima, A. A., Islam, Md. R., & Watanobe, Y. (2021). Brain-computer interface: Advancement and challenges. Sensors, 21(17). doi:10.3390/s21175746
3. Adeoye, S., & Adams, R. (2025). Neuralink’s brain-machine interfaces: A new frontier in healthcare transformation. Cognizance Journal of Multidisciplinary Studies, 5(1), 105–124. doi:10.47760/cognizance.2025.v05i01.009
4. Dadia, T., & Greenbaum, D. (2019). Neuralink: The ethical ‘rithmatic of reading and writing to the brain. AJOB Neuroscience, 10(4), 187–189. doi:10.1 080/21507740.2019.1665129
5. Zhao, W., Johnston, K. G., Ren, H. (2023). Inferring neuron-neuron communications from single-cell transcriptomics through NeuronChat. Nature Communications, 14. doi:10.1038/s41467-02336800-w
6. Drukarch, B., & Wilhelmus, M. M. (2023). Thinking about the action potential: The nerve signal as a window to the physical principles guiding neuronal excitability. Frontiers in Cellular Neuroscience, 17. doi:10.3389/fncel.2023.1232020
7. Brandman, D. M., Cash, S. S., & Hochberg, L. R. (2017). Review: Human intracortical recording and neural decoding for brain-computer interfaces. IEEE Transactions on Neural Systems and Rehabilitation Engineering, 25(10), 1687–1696. doi:10.1109/TNSRE.2017.2677443
8. Bonini, L., Rotunno, C., Arcuri, E., & Gallese, V. (2022). Mirror neurons 30 years later: Implications and applications. Trends in Cognitive Sciences, 26(9), 767–781. doi:10.1016/j. tics.2022.06.003
9. Ajrawi, S., Rao, R., & Sarkar, M. (2021). Cybersecurity in brain-computer interfaces: RFIDbased design-theoretical framework. Informatics in Medicine Unlocked, 22. doi:10.1016/j. imu.2020.100489
10. dos Santos, E. M., Cassani, R., Falk, T. H., & Fraga, F. J. (2020). Improved motor imagery brain-computer interface performance via adaptive modulation filtering and two-stage classification. Biomedical Signal Processing and Control, 57. doi:10.1016/j.bspc.2019.101812
11. Ahmed, S. F. B., Amin, M. R., & Islam, M. K. (2024). Motion artifact contaminated multichannel EEG dataset. Data in brief, 57. doi:10.1016/j. dib.2024.110994
12. Gao, X., Wang, Y., Chen, X., & Gao, S. (2021). Interface, interaction, and intelligence in generalized brain–computer interfaces. Trends in Cognitive Sciences, 25(8), 671–684. doi:10.1016/j. tics.2021.04.003
13. Lotte, F., Bougrain, L., Cichocki, A., Clerc, M., Congedo, M., Rakotomamonjy, A., & Yger, F. (2018). A review of classification algorithms for EEGbased brain–computer interfaces: A 10 year update. Journal of Neural Engineering, 15(3). doi:10.1088/1741-2552/aab2f2
14. Rao, Z., Zhang, R., He, S., Zhou, Y., Lu, Z., Li, K., & Li, Y. (2025). A once-calibration brain-computer interface to enhance convenience for continuous BCI interventions in stroke patients. IEEE Sensors Journal, 25(2), 3949–3963. doi:10.1109/ jsen.2024.3510059
15. Giles, J., Ang, K. K., Phua, K. S., & Arvaneh, M. (2022). A transfer learning algorithm to reduce brain-computer interface calibration time for long-term users. Frontiers in Neuroergonomics, 3. doi:10.3389/fnrgo.2022.837307
16. Pasqualotto, E., Matuz, T., Federici, S., Ruf, C. A., Bartl, M., Olivetti Belardinelli, M., Birbaumer, N., & Halder, S. (2015). Usability and workload of access technology for people with severe motor impairment: A comparison of brain-computer interfacing and eye tracking. Neurorehabilitation and Neural Repair, 29(10), 950–957. doi:10.1177/1545968315575611
17. Maiseli, B., Abdalla, A. T., Massawe, L. V., Mbise, M., Mkocha, K., Nassor, N. A., Ismail, M., Michael, J., & Kimambo, S. (2023). Brain-computer interface: Trend, challenges, and threats. Brain Informatics, 10(1), 20. doi:10.1186/s40708-023-00199-3
18. Veena, N., & Anitha, N. (2020). A review of non-invasive BCI devices. International Journal of Biomedical Engineering and Technology, 34(3), 205. doi:10.1504/ijbet.2020.111471
19. Armstrong, W., & Michael, K. (2020). The implications of Neuralink and brain machine interface technologies. 2020 IEEE International Symposium on Technology and Society (ISTAS), 201–203. doi:10.1109/istas50296.2020.9462223
20. Ramadan, R. A., & Vasilakos, A. V. (2017). Brain computer interface: Control signals review. Neurocomputing, 223, 26–44. doi:10.1016/j.neucom.2016.10.024
21. Caiado, F., & Ukolov, A. (2025). The history, current state and future possibilities of the non-invasive brain computer interfaces. Medicine in Novel Technology and Devices, 25, 100353. doi:10.1016/j.medntd.2025.100353
22. Rouanne, V., Costecalde, T., Benabid, A. L. (2022) . Unsupervised adaptation of an ECoG based brain–computer interface using neural correlates of task performance. Scientific Reports, 12. doi:10.1038/s41598-022-25049-w
23. Umeda, T., Isa, T., & Nishimura, Y. (2022). Temporal dynamics of the sensorimotor convergence underlying voluntary limb movement. Proceedings of the National Academy of Sciences, 119(48). doi:10.1073/pnas.2208353119
24. Fiani, B., Reardon, T., Ayres, B., Cline, D., & Sitto, S. R. (2021). An examination of prospective uses and future directions of Neuralink: The brain-machine interface. Cureus, 13(3). doi:10.7759/cureus.14192
25. Yang, D., Tian, G., Chen, J., Liu, Y., Fatima, E., Qiu, J., Malek, N. A., & Qi, D. (2025). Neural electrodes for brain-computer interface system: From rigid to soft. Biomedical Engineering Materials. doi:10.1002/bmm2.12130
26. Musk, E. (2019). An integrated brain-machine interface platform with thousands of channels. Journal of Medical Internet Research, 21(10). doi:10.2196/16194
27. Pisarchik, A. N., Maksimenko, V. A., & Hramov, A. E. (2019). From novel technology to novel applications: Comment on “An integrated brain-machine interface platform with thousands of channels” by Elon Musk and Neuralink. Journal of Medical Internet Research, 21(10). doi:10.2196/16356
28. Valle, G. (2019). The connection between the nervous system and machines: Commentary. Journal of Medical Internet Research, 21(11). doi:10.2196/16344
29. Aler, R., & Galván, I. M. (2015). Optimizing the number of electrodes and spatial filters for brain–computer interfaces by means of an evolutionary multi-objective approach. Expert Systems with Applications, 42(15–16), 6215–6223. doi:10.1016/j.eswa.2015.03.008
30. Sun, X. Y., & Ye, B. (2023). The functional differentiation of brain–computer interfaces (BCIs) and its ethical implications. Humanities and Social Sciences Communications, 10. doi:10.1057/ s41599-023-02419-x
31. Niketeghad, S., Pouratian, N. (2019). Brain machine interfaces for vision restoration: The current state of cortical visual prosthetics. Neurotherapeutics, 16, 134–143. doi:10.1007/ s13311-018-0660-1
32. Armocida, D., Garbossa, D. & Cofano, F. (2024). Letter: Ethical concerns and scientific communication on neuralink device. Neurosurgical Review, 47. doi:10.1007/s10143-024-02432-x
33. Waisberg, E., Ong, J. & Lee, A.G. (2024). Ethical considerations of Neuralink and brain-computer interfaces. Annals of Biomedical Engineering, 52, 1937–1939. doi:10.1007/s10439-024-03511-2
34. Bai, Z., Fong, K. N. K., Zhang, J. J. (2020) . Immediate and long-term effects of BCI-based rehabilitation of the upper extremity after stroke: A systematic review and meta-analysis. Journal of NeuroEngineering and Rehabilitation, 17. doi:10.1186/s12984-020-00686-2
35. Bjånes, D. A., Kellis, S., Nickl, R., Baker, B., Aflalo, T., Bashford, L., Chivukula, S., Fifer, M. S., Osborn, L. E., Christie, B., Wester, B. A., Celnik, P. A., Kramer, D., Pejsa, K., Crone, N. E., Anderson, W. S., Pouratian, N., Lee, B., Liu, C. Y., Tenore, F., Rieth, L., & Andersen, R. A. (2024). Quantifying physical degradation alongside recording and stimulation performance of 980 intracortical microelectrodes chronically implanted in three humans for 956-2246 days. doi:10.1016/j.actbio.2025.02.030
36. Leuthardt, E. C., Moran, D. W., & Mullen, T. R. (2021). Defining surgical terminology and risk for brain computer interface technologies. Frontiers in Neuroscience, 15. doi:10.3389/ fnins.2021.599549
37. Maiseli, B., Abdalla, A. T., Massawe, L. V., Mbise, M., Mkocha, K., Nassor, N. A., Ismail, M., Michael, J., & Kimambo, S. (2023). Brain-computer interface: Trend, challenges, and threats. Brain informatics, 10(1). doi:10.1186/s40708-023-00199-3
38. Hancock, P. A. (2022). Science in peril: The crumbling pillar of peer review. Theoretical Issues in Ergonomics Science, 25(2), 187–191. doi:10.1080/1 463922x.2022.2157066
39. Neziri, S., Köseoğlu, A. E., Deniz Köseoğlu, G., Özgültekin, B., & Özgentürk, N. Ö. (2024). Animal models in neuroscience with alternative approaches: Evolutionary, biomedical, and ethical perspectives. Animal Models and Experimental Medicine, 7(6), 868–880. doi:10.1002/ame2.12487
40. Parikh, P. M., & Venniyoor, A. (2024). Neuralink and brain-computer interface-exciting times for artificial intelligence. South Asian journal of cancer, 13(1), 63–65. doi:10.1055/s-0043-1774729
41. Jwa, A. S., & Poldrack, R. A. (2022). Addressing privacy risk in neuroscience data: From data protection to harm prevention. Journal of law and the Biosciences, 9(2). doi:10.1093/jlb/lsac025
42. Ochang, P., Stahl, B. C., & Eke, D. (2022). The ethical and legal landscape of brain data governance. PLOS One, 17(12). doi:10.1371/journal. pone.0273473
43. Magee, P., Ienca, M., & Farahany, N. (2024). Beyond neural data: Cognitive biometrics and mental privacy. Neuron, 112(18), 3017–3028. doi:10.1016/j.neuron.2024.09.004
44. California Consumer Privacy Act of 2018, Cal. Civ. Code § 1798.100 et. seq.
45. Isbrücker, A. (2024). There’s a basilisk in the bathwater: AI and the apocalyptic imagination. Religions, 15(5). doi:10.3390/rel15050560
46. Lavazza, A., Balconi, M., Ienca, M., Minerva, F., Pizzetti, F. G., Reichlin, M., Samorè, F., Sironi, V. A., Sosa Navarro, M., & Songhorian, S. (2025). Neuralink’s brain-computer interfaces: Medical innovations and ethical challenges. Frontiers in Human Dynamics, 7. doi:10.3389/ fhumd.2025.1553905
47. Edelman, B. J., Zhang, S., Schalk, G., Brunner, P., Müller-Putz, G., Guan, C., & He, B. (2025). Non-invasive brain-computer interfaces: State of the art and trends. IEEE Reviews in Biomedical Engineering, 18, 26–49. doi:10.1109/ rbme.2024.3449790
48. Maynard, A. D., & Scragg, M. (2019). The ethical and responsible development and application of advanced brain machine interfaces. Journal of Medical Internet Research, 21(10). doi:10.2196/16321