CROP IMPROVEMENT THROUGH MICROBIAL BIOTECHNOLOGY
Edited by Ram Prasad
Amity University, Noida, India
Sarvajeet S. Gill
MD University, Rohtak, India
Narendra Tuteja
Amity University, Noida, India ICGEB, New Delhi
Elsevier
Radarweg 29, PO Box 211, 1000 AE Amsterdam, Netherlands
The Boulevard, Langford Lane, Kidlington, Oxford OX5 1GB, United Kingdom 50 Hampshire Street, 5th Floor, Cambridge, MA 02139, United States
© 2018 Elsevier B.V. All rights reserved.
No part of this publication may be reproduced or transmitted in any form or by any means, electronic or mechanical, including photocopying, recording, or any information storage and retrieval system, without permission in writing from the publisher. Details on how to seek permission, further information about the Publisher’s permissions policies and our arrangements with organizations such as the Copyright Clearance Center and the Copyright Licensing Agency, can be found at our website: www.elsevier.com/permissions.
This book and the individual contributions contained in it are protected under copyright by the Publisher (other than as may be noted herein).
Notices
Knowledge and best practice in this field are constantly changing. As new research and experience broaden our understanding, changes in research methods, professional practices, or medical treatment may become necessary.
Practitioners and researchers must always rely on their own experience and knowledge in evaluating and using any information, methods, compounds, or experiments described herein. In using such information or methods they should be mindful of their own safety and the safety of others, including parties for whom they have a professional responsibility.
To the fullest extent of the law, neither the Publisher nor the authors, contributors, or editors, assume any liability for any injury and/or damage to persons or property as a matter of products liability, negligence or otherwise, or from any use or operation of any methods, products, instructions, or ideas contained in the material herein
Library of Congress Cataloging-in-Publication Data
A catalog record for this book is available from the Library of Congress
British Library Cataloguing-in-Publication Data
A catalogue record for this book is available from the British Library
ISBN: 978-0-444-63987-5
For information on all Elsevier publications visit our website at https://www.elsevier.com/books-and-journals
Publisher: John Fedor
Acquisition Editor: Kostas Marinakis
Editorial Project Manager: Susan Ikeda
Production Project Manager: Nilesh Kumar Shah
Cover Designer: Greg Harris
Typeset by SPi Global, India
Contributors xi
1 The Use of Microorganisms for Gene Transfer and Crop Improvement
MEHMET C. BALOGLU, MUSA KAVAS, SONGÜL GÜREL, EKREM GÜREL
1 Agrobacterium-Based Technologies 1
2 Crop Improvement Through Transgenic Technology 3
3 Virus-Induced Transient Gene Expression in Plants 8
4 Microorganisms for Crop Improvement 14 References 17
2 Actinomycetes as Potential Plant Growth-Promoting Microbial Communities
DHANANJAYA P. SINGH, HEMANT J. PATIL, RATNA PRABHA, MAHESH S. YANDIGERI, SIDDEGOWDA R. PRASAD
1 Introduction 27
2 Actinomycetes as Plant Growth Promoters 30
3 Actinomycetes for Disease Suppression 32
4 Actinomycetes for Biodegrading and Bioremediation 34
5 Production of Novel Substances 34
6 Futuristic Approaches 34 References 35
3 Microbial Genes in Crop Improvement
KRISHAN KUMAR, CHETANA AGGARWAL, SAPNA, ISHWAR SINGH, PRANJAL YADAVA
1 Introduction 39
2 Microbial Genes and Genetic Elements Deployed for Plant Transformation 40
3 Microbial Genes for Insect Resistance 42
4 Microbial Genes for Herbicide Tolerance 45
5 Microbial Genes for Modified Product Quality 45
6 Microbial Genes for Abiotic Stress Tolerance 48
7 Microbial Genes for Pathogen Resistance 49
8 Microbial Genes for Hybrid Seed Production 51
9 Public Perceptions and Biosafety Aspects of Use of Microbial Genes in Crop Improvement 52
10 Coevolution of Plants and Microbes and Presence of Microbial Genetic Elements in Native Plants 52
11 Load of Microbial Ingestions in Human Diets 53
12 Conclusion 53 Acknowledgments 54 References 54
4 Microbial Transformations Implicit With Soil and Crop Productivity in Rice System
VAVILALA R. RAO
1 Introduction 57
2 Microbial Niches in Submerged Soils 58
3 Major Microflora Associated With Rice Soil 59
4 Sustainability of Rice Soil Ecosystems 60
5 Microbial Involvement in Sustainability 62
6 Altering Anaerobic and Aerobic Interface and Pesticide Biodegradation 64
7 Implication of Microbial Methane Production From Flooded Soil 64
8 Mitigation Options for Reducing Methane Emission From Flooded Rice 66
9 Conclusions 70 References 70
5 Application of Microbial Biotechnology in Food Processing
SUBRATA N. BHOWMIK, RAMABHAU T. PATIL
1 Introduction 73
2 Current Status of Microbial Biotechnology in Food Processing 75
3 Conclusion 98 References 99 Further Reading 106
6 Innate Immunity Engaged or Disengaged in Plant-Microbe Interactions
SRIDHAR RANGANATHAN
1 Beginning of Molecular Basis of Plant-Microbe Interactions 107
2 Plant-Pathogen Interactions 108
3 Innate Immunity 108
4 Mutualistic Interactions 120
5 Epilogue 130 References 131 Further Reading 144
7 Novel Strategies for Engineering Resistance to Plant Viral Diseases
MEENAKSHI DANGWAL, SHIVARAJ M. MATHAD, BASAVAPRABHU L. PATIL
1 Introduction 145
2 Natural and Engineered Resistance Against Plant Viruses 147
3 Mechanisms of Resistance to Plant Viruses 151
4 RNA Silencing Pathways 155
5 Genome Editing Tools to Combat Plant Viruses 162
6 Prospects 164 Acknowledgments 164 References 164 Further Reading 174
8 Molecular Characterization of Sugarcane Viruses and Their Diagnostics
RASAPPA VISWANATHAN, BALASUBRAMANIAN PARAMESWARI, KATHIRVEL NITHYA
1 Introduction 175
2 Mosaic 176
3 Leaf Fleck 181
4 Yellow Leaf Disease 184
5 Detection and Diagnosis of Mixed Infections 186
6 Conclusion 187 Acknowledgments 188 References 188
9 Cyanobacterial Biodiversity and Biotechnology: A Promising Approach for Crop Improvement
SHIVAM YADAV, RUCHI RAI, ALOK K. SHRIVASTAVA, PRASHANT K. SINGH, SONIA SEN, ANTRA CHATTERJEE, SHWETA RAI, SHILPI SINGH, LAL C. RAI
1 Introduction 195
2 Crop Yield Constraint, Population Increase and Food Security 196
3 Application of Cyanobacteria in Crop Improvement and Sustainable Agriculture 200
4 Conclusion 211 Acknowledgments 211 References 211 Further Reading 219
10 Pseudomonas fluorescens: A PlantGrowth-Promoting Rhizobacterium (PGPR) With Potential Role in Biocontrol of Pests of Crops
BALIAH V. DAVID, GOVINDAN CHANDRASEHAR, PAMILA N. SELVAM
1 Introduction 221
2 General Characteristics of Pseudomonas fluorescens 222
3 Plant Growth Promoting Properties of Pseudomonas 222
4 Mechanisms of Plant Growth Promotion by Pseudomonas 223
5 Induction of Systemic Resistance by PGPR Against Diseases, Insect and Nematode Pests 225
6 Synergistic Effect of PGPR Strain Mixtures 227
7 PGPR as Endophytes 227
8 Mode of Action of Pseudomonas Against Fungal Pathogens 228
9 Plant Diseases Control by P. fluorescens 229
10 Interaction of P. fluorescens With Chemical Pesticides 232
11 Formulation Characteristics of Biopesticides 233
12 Approved Uses of P. fluorescens Formulations in India 233
13 Regulation for Biopesticides 236
14 Data Requirements for Biopesticides
Registration 236
15 Regulatory Mechanisms for Biopesticides 237
16 Factors Affecting Growth of Biopesticides 238
17 Future Issues and Research Needs in Biopesticides 238 References 239 Further Reading 243
11 Crop Improvement Through Microbial Technology: A Step Toward Sustainable Agriculture
PANKAJ BHATT, TAPAN K. NAILWAL
1 Introduction 245
2 Crop Production Scenario in World 246
3 Crop Production in India 247
4 Microbial Technology for Crop Production 247
5 Microbial Biotechnology for Crop Production 249
6 Conclusion 250 Acknowledgment 251 References 251 Further Reading 253
12 Microbial Technologies for Sustainable Crop Production
CHITTRANJAN BHATIA, PRASUN K. MUKHERJEE
1 Introduction 255
2 Origin of Farming 256
3 Urgent Need to Increase Sustainable Crop Productivity 256
4 Undesired Effects of Increased Inputs of Chemical Fertilizers and Pesticides 257
5 Rhizosphere Microbial Diversity 257
6 Crop Production as an Energy Harvesting Process 257
7 Root Exudates Support Microbial Populations in the Rhizosphere 258
8 New Techniques 258
9 Using Microbial Diversity for Enhanced Crop Production 258
10 Registration and Commercial Issues 259
11 Challenges of Microbial Products 260
12 Conclusions and Outlook for the Future 260 References 261
13 Trichoderma: Its Multifarious Utility in Crop Improvement
MUJEEBUR R. KHAN, FAYAZ A. MOHIDDIN
1 Introduction 263
2 Taxonomy of Trichoderma 265
3 Factors Influencing Activity of Trichoderma
Species 271
4 Mechanism of Action of Trichoderma
Species 272
5 Plant Growth Promotion by Trichoderma
Species 276
6 Conclusion and Future Prospects 282 References 282 Further Reading 291
14 Microbe-Mediated Enhancement of Nitrogen and Phosphorus Content for Crop Improvement
MANOJ NATH, DEEPESH BHATT, MEGHA D. BHATT, RAM PRASAD, NARENDRA TUTEJA
1 Introduction 293
2 Plant Growth Promoting Rhizobacteria (PGPR) Mediated N and P Enhancement During Plant Microbe Interaction 294
3 AMF and Enhancement of N and P in Plants 298
4 Conclusions 301 References 301
15 Microbiome in Crops: Diversity, Distribution, and Potential Role in Crop Improvement
AJAR N. YADAV, VINOD KUMAR, HARCHARAN S. DHALIWAL, RAM PRASAD, ANIL K. SAXENA
1 Introduction 305
2 Isolation and Characterization of Crop Microbiomes 307
3 Diversity and Distribution of Crop Microbiomes 310
4 Beneficial Role of Microbes in Crop Improvement 316
5 Conclusion and Future Scope 322 References 323
16 Plant Growth-Promoting Rhizobacteria (PGPR): Perspective in Agriculture Under Biotic and Abiotic Stress
AJAY KUMAR, VIPIN K. SINGH, VIJAY TRIPATHI, PREM P. SINGH, AMIT K. SINGH
1 Introduction 333
2 Stress Conditions Affecting Plant Growth 335
3 Role of PGPR Against Biotic Stress 335
4 Role of PGPR in Mitigation of Draught and Salinity Stress 337
5 Role of PGPR in Phytoremediation of Metal Contaminated Sites 338
6 Conclusions 339 References 340 Further Reading 342
17 Rhizosphere Metabolite Profiling: An Opportunity to Understand Plant-Microbe Interactions for Crop Improvement
AMIT VERMA, SATENDRA KUMAR, HEMANSI, GOVIND KUMAR, JITENDRA K. SAINI, RUCHI AGRAWAL, ALOK SATLEWAL, MOHAMMAD W. ANSARI
1 Introduction 343
2 Plant Microbial Environment and Root Exudates 344
3 Rhizosphere Metabolites 347
4 Transcriptomics in Rhizosphere Study 348
5 Metabolomics in Rhizosphere Study 351
6 Future Prospects and Conclusion 356 References 356 Further Reading 361
18 Phosphate-Solubilizing Pseudomonads for Improving Crop Plant Nutrition and Agricultural Productivity
BALA RATHINASABAPATHI, XUE LIU, YUE CAO, LENA Q. MA
1 Phosphorus Nutrition for Crop Production 363
2 Phosphate Solubilization by Rhizosphere Microorganisms 364
3 Mechanisms of Phosphorus-Solubilizing Bacteria 366
4 Use of Pseudomonads in Agriculture Products 369 Acknowledgments 370
References 370 Further Reading 372
19 Targeted Genome Editing for Crop Improvement in Post Genome-Sequencing Era
CHANDRA P. SINGH, NAVNEET S. CHAUDHARY, BASKARAN KANNAN, RATNA KARAN
1 Introduction 373
2 Basic Mechanism of Genome Editing 374
3 Double Strand Breaks (DSBs) and Repairing Pathways 374
4 Sequence Specific Nucleases 376
5 Application of Sequence Specific Nucleases in Plants 382 References 385 Further Reading 389
20 Endophytic Microorganisms: Their Role in Plant Growth and Crop Improvement
MANJU SHARMA, REKHA KANSAL, DINESH SINGH
1 Introduction 391
2 Mode of Transmission 392
3 Colonization 393
4 Types of Endophytes 395
5 Role of Endophytes in Crop Improvement 399
6 Conclusion 405 References 405
21 Microbes in Crop Improvement: Future Challenges and Perspective
KASHYAP K. DUBEY, PUNIT KUMAR
1 Introduction 415
2 Microbes to as Biocontrol Control Plant
Disease Control 416
3 Antagonism 417
4 Competition 417
5 Induced Resistance 419
6 Microbes as Biofertilization (Improved Plant Nutrient Availability) 419
7 Nitrogen Fixation 419
8 Phosphate Solubilization 420
9 Plant Growth Promoting Hormones 420
10 Enhanced Stress Tolerance 420
11 Use and Commercialization of Plant Growth Promoting Rhizobacteria 421 References 423
22 Plant-Microbe Interaction and Genome Sequencing: An Evolutionary Insight
KRISHNA
K. SHARMA, DEEPTI SINGH, BIJENDER SINGH, SARVAJEET S. GILL, AMARJEET SINGH, BHUVNESH
SHRIVASTAVA
1 Introduction 427
2 Host-Microbe and Microbe-Microbe Interaction 428
3 Clavicipitaceous Endophytes and Their Role 430
4 Effects on Disease Resistance and Susceptibility 431
5 Roles in Plant Ecophysiology 431
6 Class 2 Endophytes and Their Roles 431
7 Symbiotically Derived Benefits to Endophytes 432
8 Endophyte-Conferred Fitness Benefits and Ecological Adaptations of Plants 432
9 Mechanisms of Stress Tolerance 433
10 Class 3 Endophytes 433
11 Class 4 Endophytes 434
12 Host-Microbe Interaction and Plant Immunity 434
13 Microbial Genome and Plant-Microbe Interaction 436
14 Lifestyle Transition in Plant Pathogens 438
15 Genome Evolution in Bacterial and Fungal Plant Pathogen 439
16 Comparative Genomics to Study Plant-Pathogen Coevolution 440
17 Metagenomic Analysis: Metadata of Obligate Biotrophs 442
18 Conclusion and Future Prospects 443 Acknowledgment 443 References 443 Further Reading 449
23 Crop Breeding Using CRISPR/Cas9
SHIGEO S. SUGANO, KEISHI OSAKABE, YURIKO OSAKABE
1 Introduction 451
2 CRISPR/Cas9 452
3 Molecular Breeding in Crop Plants Using the CRISPR/Cas9 System 454
4 Potential Problems With Crop Breeding By Genome Editing 457
5 Conclusion 460 References 460
24 Bioprospecting PGPR Microflora by Novel Immunobased Techniques
RUCHI AGRAWAL, AMIT VERMA, ALOK SATLEWAL
1 Introduction 465
2 Plant Growth Promoting Rhizobacteria (PGPR) 466
3 Bioprospecting Plant Growth Promoting Rhizobacteria 467
4 Recent Techniques for PGPR Detection/ Characterization 468
5 Molecular Techniques 468
6 Immunological Methods 471
7 Conclusion 474 References 474
Index 479
Contributors
Chetana Aggarwal Indian Council of Agricultural Research—Indian Institute of Maize Research, New Delhi, India
Ruchi Agrawal Govind Ballabh Pant University of Agriculture and Technology (GBPUAT), Pantnagar, India
Mohammad W. Ansari Zakir Hussain College, Delhi University, New Delhi, India
Mehmet C. Baloglu Kastamonu University, Kastamonu, Turkey
Chittranjan Bhatia Mumbai, India
Pankaj Bhatt Dolphin Institute of Biomedical and Natural Sciences, Dehradun, Uttarakhand, India
Deepesh Bhatt Shree Ramkrishna Institute of Computer Education and Applied Sciences, Affiliated to Veer Narmad South Gujarat University, Surat, India
Megha D. Bhatt GSFC AgroTech Ltd., Gujarat State Fertilizers & Chemicals Ltd., Vadodara, India
Subrata N. Bhowmik ICAR Research Complex for NEH Region, Lembucherra, India
Yue Cao Nanjing University, Nanjing, China
Govindan Chandrasehar International Institute of Biotechnology and Toxicology (IIBAT), Padappai, India
Antra Chatterjee Banaras Hindu University, Varanasi, India
Navneet S. Chaudhary University of Rajasthan, Jaipur, Rajasthan, India
Meenakshi Dangwal ICAR-National Research Centre on Plant Biotechnology, IARI, New Delhi, India
Baliah V. David International Institute of Biotechnology and Toxicology (IIBAT), Padappai, India
Harcharan S. Dhaliwal Eternal University, Sirmour, India
Kashyap K. Dubey Central University of Haryana, Mahendergarh; Maharshi Dayanand University, Rohtak, Haryana, India
Sarvajeet S. Gill Maharshi Dayanand University, Rohtak, India
Songül Gürel Sugar Institute, Ankara, Turkey
Ekrem Gürel Abant Izzet Baysal University, Bolu, Turkey
Hemansi Department of Microbiology, Central University of Haryana, Mahendergarh, Haryana, India
Baskaran Kannan University of Florida, Gainesville, FL, United States
Rekha Kansal Indian Agricultural Research Institute, New Delhi, India
Ratna Karan University of Florida, Gainesville, FL, United States
Musa Kavas Ondokuz Mayıs University, Samsun, Turkey
Mujeebur R. Khan Aligarh Muslim University, Aligarh, India
Krishan Kumar Indian Council of Agricultural Research—Indian Institute of Maize Research, New Delhi, India
Vinod Kumar Eternal University, Sirmour, India
Ajay Kumar Banaras Hindu University, Varanasi, India
Satendra Kumar Govind Ballabh Pant University of Agriculture and Technology (GBPUAT), Pantnagar, India
Govind Kumar Govind Ballabh Pant University of Agriculture and Technology (GBPUAT), Pantnagar, India
Punit Kumar Maharshi Dayanand University, Rohtak, Haryana, India
Xue Liu Nanjing University, Nanjing, China
Lena Q. Ma University of Florida, Gainesville, FL, United States; Nanjing University, Nanjing, China
Shivaraj M. Mathad ICAR-National Research Centre on Plant Biotechnology, IARI, New Delhi, India
Fayaz A. Mohiddin SKUAST-K, Srinagar, India
Prasun K. Mukherjee Bhabha Atomic Research Centre, Mumbai, India
Tapan K. Nailwal Kumaun University, Nainital, Uttarakhand, India
Manoj Nath Amity Institute of Microbial Technology, Amity University, Noida, India
Kathirvel Nithya ICAR-Sugarcane Breeding Institute, Coimbatore, India
Keishi Osakabe Tokushima University, Tokushima, Japan
Yuriko Osakabe Tokushima University, Tokushima, Japan
Balasubramanian Parameswari ICARSugarcane Breeding Institute Regional Centre, Karnal, India
Hemant J. Patil Institute of Soil, Water and Environmental Sciences, Volcani Center, Agricultural Research Organization, Bet Dagan, Israel
Ramabhau T. Patil Benevole Welfare Society for Post Harvest Technology, Bhopal, India
Basavaprabhu L. Patil ICAR-National Research Centre on Plant Biotechnology, IARI, New Delhi, India
Ratna Prabha ICAR—National Bureau of Agriculturally Important Microorganisms, Indian Council of Agricultural Research, Maunath Bhanjan, India
Siddegowda R. Prasad ICAR—Indian Institute of Seed Science, Indian Council of Agricultural Research, Maunath Bhanjan, India
Ram Prasad Amity Institute of Microbial Technology, Amity University, Noida, India
Ruchi Rai Banaras Hindu University, Varanasi, India
Shweta Rai Banaras Hindu University, Varanasi, India
Lal C. Rai Banaras Hindu University, Varanasi, India
Sridhar Ranganathan Independent Researcher, Chennai, Tamil Nadu, India
Vavilala R. Rao Central Rice Research Institute, Cuttack, India
Bala Rathinasabapathi University of Florida, Gainesville, FL, United States
Jitendra K. Saini Department of Microbiology, Central University of Haryana, Mahendergarh, Haryana, India
Sapna Indian Council of Agricultural Research—Indian Institute of Maize Research, New Delhi, India
Alok Satlewal Govind Ballabh Pant University of Agriculture and Technology (GBPUAT), Pantnagar, India
Anil K. Saxena ICAR-National Bureau of Agriculturally Important Microorganisms, Mau, India
Pamila N. Selvam International Institute of Biotechnology and Toxicology (IIBAT), Padappai, India
Sonia Sen Banaras Hindu University, Varanasi, India
Manju Sharma Amity University Haryana, Manesar, India
Krishna K. Sharma Maharshi Dayanand University, Rohtak, India
Alok K. Shrivastava Banaras Hindu University, Varanasi, India
Bhuvnesh Shrivastava Panacea Biotech Limited, New Delhi, India
Dhananjaya P. Singh ICAR—National Bureau of Agriculturally Important Microorganisms, Indian Council of Agricultural Research, Maunath Bhanjan, India
Ishwar Singh Indian Council of Agricultural Research—Indian Institute of Maize Research, New Delhi, India
Prashant K. Singh Banaras Hindu University, Varanasi, India
Shilpi Singh Banaras Hindu University, Varanasi, India
Vipin K. Singh Banaras Hindu University, Varanasi, India
Prem P. Singh Banaras Hindu University, Varanasi, India
Amit K. Singh Banaras Hindu University, Varanasi, India; Agricultural Research Organization, Volcani Center, Bet-Dagan, Israel
Chandra P. Singh University of Rajasthan, Jaipur, Rajasthan, India
Dinesh Singh Indian Agricultural Research Institute, New Delhi, India
Deepti Singh Maharshi Dayanand University, Rohtak, India
Bijender Singh Maharshi Dayanand University, Rohtak, India
Amarjeet Singh University of Delhi, New Delhi, India
Shigeo S. Sugano Ritsumeikan University, Kyoto, Japan
Vijay Tripathi Sam Higginbottom University of Agriculture Technology and Sciences, Allahabad, India
Narendra Tuteja Amity Institute of Microbial Technology, Amity University, Noida; ICGEB, New Delhi, India
Amit Verma Gujarat Agricultural University, SK Nagar, India
Rasappa Viswanathan ICAR-Sugarcane Breeding Institute, Coimbatore, India
Shivam Yadav Banaras Hindu University, Varanasi, India
Ajar N. Yadav Eternal University, Sirmour, India
Pranjal Yadava Indian Council of Agricultural Research—Indian Institute of Maize Research, New Delhi, India; Stanford University, Stanford, CA, United States
Mahesh S. Yandigeri ICAR—National Bureau of Agricultural Insect Resources (NBAIR), Hebbal, India
The Use of Microorganisms for Gene Transfer and Crop Improvement
Mehmet C. Baloglu*, Musa Kavas†, Songül Gürel‡, Ekrem Gürel§
*Kastamonu University, Kastamonu, Turkey †Ondokuz Mayıs University, Samsun, Turkey ‡Sugar Institute, Ankara, Turkey §Abant Izzet Baysal University, Bolu, Turkey
1 AGROBACTERIUM-BASED TECHNOLOGIES
1.1 Gene Transfer Through Agrobacterium Tumefaciens
Steady increase in world population is forcing the scientists to increase agriculture production. Earlier crop improvement techniques based on hybridization of genotypes have had different characteristics. However, crop improvement through conventional breeding methods requires a wide gene pool in genetically close plant species. By discovery of recombinant DNA technology, genetic engineering has become the most widely used tool in crop improvement. One of the most important developments in plant biotechnology is the ability of transferring foreign genes into plant genome. The gene transfers between crops and other unrelated organisms, which have potential candidate genes, lead to the production of improved varieties in terms of yield and resistance to disease, pest, and herbicides. Many different genetic transformation techniques were developed to obtain transgenic plants over the last three decades. Among various gene delivery techniques, Agrobacterium-mediated genetic transformation and particle bombardment are the most widely used for the genetic engineering of plants. Low copy number transgenesis and the production of high-quality transgenic plants are the most important advantages of Agrobacterium-mediated gene transfer when compared to the particle bombardment or biolistic (Dai et al., 2001). The first genetically modified plant was produced in 1982 with A. tumefaciens by using tobacco leaf tissue (Fraley et al., 1983). Up to now, gene transfer with disarmed (nontumorigenic) Agrobacterium strains has been achieved by using more than 120 plant species such as maize, wheat, soybean, cotton, tobacco, and rice (Abhishek et al., 2016). A. tumefaciens, a member of Rhizobiaceae family, has been used for genetic transformation studies in plants. This ubiquitous gram-negative soil bacterium has an
ability to transfer its segment of plasmid (Ti-Ri plasmid) surrounded by repeated nucleotides into plant genome naturally. The typical Ti plasmid, with a crucial role in crown gall disease, is about 200 kb. Naturally grown Agrobacterium cells carry two types of gene on T-DNA region. The first one known as oncogenic genes includes auxin and cytokinin genes. The others are responsible for opine and agropine synthesis in infected plant tissues (Gustavo et al., 1998). The proteins coded by vir (virulence) genes carried out the transfer of T-DNA region into the plant cells. Phenolic substances released from wounded plant tissues induce the activation of vir genes located in tumor-inducing (Ti) plasmid of A. tumefaciens. There are about 30 genes in A. tumefaciens vir regulon, and about 20 of them are required for tumor formation in plant tissues (Gelvin, 2003). This regulon consists of at least six operon (VirA, VirB, VirC, VirD, VirG, and VirE) required for single-stranded T-DNA generation and transfer into the host plant cell genome (Fig. 1) (Gustavo et al., 1998; Zupan and Zambryski, 1997).
The improvement of plants through the gene transfer mainly relies on the tissue-culture response of genotypes or species. In order to generate transgenic plants, suitable transformation methods and a robust regeneration protocol are required. By this context, some of the species and explants may not be suitable for Agrobacterium-mediated gene transfer. Especially, monocotyledon plant species are recalcitrant to Agrobacterium-mediated transformation. These groups of plants are not naturally infected by A. tumefaciens due to the lack of phenolic substances required for induction of vir genes. By using artificial phenolic substances and hypervirulent strain, monocot plants such as cereal can be transformed by A. tumefaciens. Another
FIG. 1 Single-stranded T-DNA generation and transfer into the host plant cell genome.
group of plants also cannot be genetically engineered because of their low regeneration potential. As previously mentioned, the production of transgenic plants requires tissue- culture steps. Recently, tissue-culture-independent methods have been demonstrated to work in a limited number of plant species. One of the most promising tissue-culture-independent methods is called floral-dip transformation. Arabidopsis thaliana plant can efficiently be transformed by using this technique (Feldmann and Marks, 1987). This technique, which removes the need for tissue culture, has been successfully applied to other plants such as soybean, radish, tomato, brinjal, and snake gourd (Hu and Wang, 1999; Curtis and Nam, 2001; Park et al., 2005; Yasmeen et al., 2009; Subramanyam et al., 2015).
1.2 Gene Transfer Through Agrobacterium Rhizogenes
The other important phytopathogens have gene transfer ability, and the cause to hairy root disease is A. rhizogenes (Gelvin, 2009). The A. rhizogenes-mediated transformation characterized by hairy root formation takes place by transferring T-DNAs from the Ri plasmid into plant cell (Tepfer and Cassedelbart, 1987). The most prominent characteristic of hairy roots induced by A. rhizogenes is that they can able to grow rapidly in the absence of exogenous plant growth regulators (Collier et al., 2005). Testing of gene functions in a short period by using stable transgenic tissues is the most important advantage of A. rhizogenes-mediated gene transfer (Kim et al., 2002). Because of this valuable property, A. rhizogenes-mediated gene transfer has turned into a powerful tool for gene functional and root biology studies (Cao et al., 2009). There are many plant species; some of them are recalcitrant to A. tumefaciens-mediated gene transfer that has been transformed by A. rhizogenes (Georgiev et al., 2007). In this context, soybean and tobacco plants were transformed to analyze gene function (Wang et al., 2016; Hao et al., 2011). In addition to the gene function analyses, A. rhizogenes-mediated gene transfer is generally used for the excess production of plant chemicals and therapeutic agents (Yao et al., 2016; Kiani et al., 2016).
1.3 Non-Agrobacterium-Based Technologies
Over 10 years ago, Broothaerts et al. (2005) have reported that there is non-Agrobacterium plant-associated bacterial species such as Sinorhizobium meliloti, Mesorhizobium loti, and Rhizobium NGR 234 that could transform A. thaliana. Although there are a limited number of studies reporting the successful transformation event by using these species, Wendt et al. (2011) and Rathore et al. (2016) have demonstrated that Ensifer adhaerens strain OV14 has the ability to transform potato and oilseed rape.
2 CROP IMPROVEMENT THROUGH TRANSGENIC TECHNOLOGY
2.1 Herbicide Resistant Transgenic Plants
The use of genetic engineering techniques to develop glyphosate-resistant (GR) crops was a scientific discovery that leads to revolutionizing the weed management strategies (Green, 2012).
Glyphosate (N-(27)-glycine) is a powerful and most widely used broad-spectrum herbicide targeting the shikimate pathway enzyme 5-enolpyruvylshikimate 3-phosphate (EPSP) synthase (Steinrücken and Amrhein, 1980). Up till now, many genes have been shown to provide a glyphosate resistance effect to different cells (Stalker et al., 1985; Comai et al., 1985; Yu et al., 2015; Ye et al., 2001; Zhou et al., 1995; Padgette et al., 1991). By using these genes, various herbicidetolerant transgenic plants have been generated (Table 1). Among these genes, the CP4-EPSPS gene, isolated from naturally glyphosate-resistant Agrobacterium strain CP4, has been widely used to produce commercially available herbicide-resistant transgenic plants (Dill et al., 2008). The CP4-EPSPS gene codes glyphosate-insensitive form of EPSP synthase enzyme. Since weed control with classical tools was time-consuming and costly, the usage of GR crops made weed management easy, efficient, economical, and environmentally compatible. The first herbicideresistant transgenic plant was produced in 1986 by introducing EPSPS gene into soybean cells (Shah et al., 1986). By this context, natural or codon-optimized EPSPS gene has been introduced into several plant species including rice, maize, bent grass, cotton, sugar beet, lettuce, and cotton (Mannerlof et al., 1997; Nida et al., 1996; Nagata et al., 2000; Cerny et al., 2010; Lee et al., 2011; Sun et al., 2015; Chhapekar et al., 2015).
TABLE 1 Important Transgenes/Transgene Products Being Used for Engineering Crop Plants Possessing Herbicide Resistance
Transgene(s) Source Plant Species Target Herbicide Reference
Bar Streptomyces hygroscopicus Tobacco, potato, tomato Phosphinothricin and bialaphos
De Block et al. (1987)
Bar Streptomyces hygroscopicus Lotus japonicus Phosphinothricin Lohar et al. (2001)
Bxn Klebsiella ozaenae Tobacco Bromoxynil (3,5-dibromo4-hydroxybenzonitrile) Stalker et al. (1988)
Bxn Klebsiella ozaenae Trifolium subterraneum L. Bromoxynil (3,5-dibromo4-hydroxybenzonitrile) Dear et al. (2003)
PgrA Ochrobactrum anthropi Tobacco Paraquat Jo et al. (2004)
Bar Streptomyces hygroscopicus Sweet potato Glufosinate Choi et al. (2007)
MxPPO Myxococcus xanthus Tall fescue Oxyfluorfen, acifluorfen Lee et al. (2008)
Bar Streptomyces hygroscopicus Ipomoea batatas Phosphinothricin and bialaphos Zang et al. (2009)
AtDHAR1 Arabidopsis thaliana Potato Methylviologen Eltayeb et al. (2011)
G6/EPSSPS Pseudomonas putida Rice Glyphosate Te et al. (2011)
G2/EPSSPS and GAT Pseudomonas fluorescens Bacillus licheniformis Soybean Glyphosate Guo et al. (2015)
DAAO Bradyrhizobium japonicum Arabidopsis Glyphosate Han et al. (2015)
Bar Streptomyces hygroscopicus Salvia miltiorrhiza Phosphinothricin Liu et al. (2015a)
Dehd Rhizobium sp. RC1 Nicotiana benthamiana Mohamed et al. (2016)
These plants have more cultivating area each year because they are making the management with weeds both easier and cheaper. This is especially the case for soybean, which is the most widely grown herbicide-tolerant plant in the world. Glyphosate-resistant soybeans represented 50% of all herbicide-resistant crops and about 80% of all globally cultivated soybeans in 2014 (James, 2015).
In addition to EPSPS gene, bar, dehd, daao, dhar1, pat, ppo, cryp1a, pgra, bxn, gat, and gst27 genes were successfully transferred into plant cell, and various herbicide-tolerant transgenic plants were obtained (Table 1). In this context, another important gene was called bar that was isolated from Streptomyces hygroscopicus. Phosphinothricin acetyltransferase (PAT) enzyme coding by bar gene has an ability to convert phosphinothricin into a nontoxic acetylated form (Gordonkamm et al., 1990). Transgenic plants have been obtained by transferring this gene to many plants including sorghum, cowpea, cotton, soybean, salvia, grape, sugar beet, apricot, and sweet potato during the last 30 years (Liu et al., 2015a, 2014; Ilori and Pellegrineschi, 2011; Petri et al., 2015; Metwali et al., 2016; Do et al., 2016; Li et al., 2009; Zang et al., 2009; Mishutkina et al., 2010).
2.2 Insect Resistant Transgenic Plants
One of the most important abiotic stress factors reducing agricultural productivity is pests. So, the second important trait introduced by Agrobacterium-mediated gene transfer into plant cell was insect resistance. There are two main approaches for the production of genetically engineered insect-resistant plants. In the context of the first approach, insect-resistant transgenic plants are generally obtained through the transferring of genes encoding crystal toxin proteins (Cry proteins) from Bacillus thuringiensis. These proteins inactivate their targets through the affecting guts. Cry genes code resistance in plants against a variety of insects belonging to Lepidoptera (Zhao et al., 2014), Coleoptera (Tohidfar et al., 2013), Hemiptera (Rausch et al., 2016), and Diptera (Andrews et al., 1987). The first example of insect-resistant transgenic plant was transgenic tobacco plant produced through the introduction of bt genes by using A. tumefaciens (Hilder et al., 1987), although the first commercially available bt transgenic plant, a transgenic maize generated for controlling corn borer (Ostrinia nubilalis), was produced using biolistic method. The number of bt transgenic species produced via Agrobacterium-mediated gene transfer has dramatically increased (Narva et al., 2013). Important plant species including maize, rice, potato, cotton, tomato, alfalfa, and chickpea have been transformed with bt genes by using A. tumefaciens (Table 2). GM crops with bt genes were globally planted over 35 million hectares in 13 different countries in 2014, and they constitute 15% of all GM crops (James, 2015).
In another strategy, other insecticidal genes from a variety of organism including plant and bacteria were transferred into plant cell. These are proteinase inhibitors, lectins, amylase inhibitors, etc. (Tran et al., 1997; Ishimoto et al., 1995; Tamayo et al., 2000; Rao et al., 1998; Christeller et al., 2002).
2.3 Nutritional Improvement
After the successful use of recombinant DNA techniques in the development of plants, improving the nutritional quality of food crops has become an important target. Genes from
TABLE 2 Important Transgenes/Transgene Products Being Used for Engineering Crop Plants Possessing Insect Resistance
Transgene(s) Source
Cry3B
Cry1A (c)
Cry2A-1Ac-gna
Cry1Ac–2A
Cry1Ab and cry1Ac
Cry1Ac9 and cry9Aa2
Cry1C
Cry1EC
Plant Species Target Insects Reference
Bacillus thuringiensis Brinjal Fruit borer Iannacone et al. (1997)
Bacillus thuringiensis Canola Lepidoptera Halfhill et al. (2001)
Bacillus thuringiensis Rice Rice weevil, rice hispa Maqbool et al. (2001)
Bacillus thuringiensis Rice
Bacillus thuringiensis Indica rice (Oryza sativa L.)
Yellow stem borer, rice leaf folder Bashir et al. (2004)
Yellow stem borer and sap-sucking insects Ramesh et al. (2004)
Bacillus thuringiensis Potato Phthorimaea operculella Meiyalaghan et al. (2006)
Bacillus thuringiensis Japonica rice Tryporyza incertulas Walker, Chilo suppressalis Walker, Cnaphalocrocis medinalis Guenec Ye et al. (2009))
Bacillus thuringiensis Cotton Spodoptera litura Kumar et al. (2009)
Cry2Ab Bacillus thuringiensis Tomato Lepidoptera, Phthorimaea operculella, Helicoverpa armigera, Saker et al. (2011)
Cry2Aa
Cry3a
Bacillus thuringiensis Chickpea Lepidoptera Mehrotra et al. (2011)
Bacillus thuringiensis Alfalfa Coleoptera Tohidfar et al. (2013))
Cry1Ab and vip3H Bacillus thuringiensis Rice Sogatella furcifera Lu et al. (2014)
Cry1Ah Bacillus thuringiensis Maize Ostrinia furnacalis Li et al. (2014)
Cry1Ah Bacillus thuringiensis Maize Corn borer Sun et al. (2015)
Cry3A
Bacillus thuringiensis Potato Leptinotarsa decemlineata Say, CPB Mi et al. (2015)
different sources were transferred into plant cell to change the nutritional content of plants. For instance, phytoene synthase (psy) and lycopene β-cyclase (β-lcy) genes from Narcissus pseudonarcissus were transferred into rice genome by using Agrobacterium-mediated gene transfer to increase vitamin A content (Beyer et al., 2002). Likewise, genetic engineering of soybean plant was carried out with A. tumefaciens including RNAi construct for β-subunit gene in 7S globulin protein to decrease antinutritional effects (Qu et al., 2016). Other good examples for improved nutritional value of crops were transgenic soybean and maize with an increased content of β-carotene and lysine, respectively (Kim et al., 2012; Liu et al., 2015b). The seeds of transgenic soybean plants had ~62-fold higher β-carotene than nontransgenic seeds (Kim et al., 2012).
2.4 Abiotic Stress Tolerance
Drought, salinity, and cold are the most important environmental stresses decreasing the agricultural production in all over the world. To increase the productivity, it is necessary
to increase tolerance against the environmental stresses. To generate abiotic stress-tolerant crops, a combinatorial approach consisting of plant biotechnology, genetics, and breeding is required (Wani et al., 2016). Plants can be genetically engineered to improve abiotic stress tolerance. Many organisms have special genes that the expression pattern significantly changed during the environmental stresses. Some of these genes have been cloned and transformed into plants by using Agrobacterium-mediated gene transfer (Table 3). For instance, for salt tolerance, P5CS, NHX1, CMO, and MYB genes have been transferred into potato, rice, alfalfa, and A. thaliana (Hmida-Sayari et al., 2005; Zhang et al., 2012; Wu et al., 2010; Li et al., 2016). Likewise, MYC-type ICE1-like transcription factor, SlICE1a, was isolated from tomato and transferred into tobacco genome. Resulted transgenic tobacco plants showed tolerance to salt, drought, and cold (Feng et al., 2013). Recently, scientist has paid more attention to WRKY and DREB transcription-factor-coding genes to generate osmotic stress-tolerant transgenic plants (Wei et al., 2016; Rushton et al., 2012; Fan et al., 2016). WRKY transcription factors in plants have roles in abiotic stress tolerance and development and immune system response (Zhang et al., 2016). Another immune system protein is osmotin, which has also abiotic stress tolerance in plants. Osmotin-coding genes were transferred into a variety of plant species including tobacco, tomato, carrot, and soybean by using Agrobacterium-mediated gene transfer (Weber et al., 2014; Annon et al., 2014; Kumar et al., 2016; Viktorova et al., 2017).
TABLE 3 Important Transgenes/Transgene Products Being Used for Engineering Crop Plants Against Abiotic stresses
Transgene(s) Source Plant Species Trait Reference
Pyrroline-5-carboxylate synthetase (P5CS)
Arabidopsis thaliana Potato Salt tolerance Hmida-Sayari et al. (2005)
CBF3/DREB1A and ABF3 Arabidopsis thaliana Rice Osmotic stress tolerance Zhao et al. (2007)
Choline monooxygenase (CMO) Salicornia europaea Tobacco Salt tolerance Wu et al. (2010)
CsWRKY46 Cucumis sativus Arabidopsis thaliana Cold tolerance Zhang et al. (2016)
Betaine aldehyde dehydrogenase (BADH) Spinacia oleracea Potato Salt and drought tolerance Zhang et al. (2011)
TaNHX2 Triticum aestivum Alfalfa Salt tolerance Zhang et al. (2012)
Mannitol-1-phosphate dehydrogenase (mtlD)
Escherichia coli Peanut Salt and osmotic stress tolerance Bhauso et al. (2014)
Choline oxidase gene (codA) Arthrobacter globiformis Sweet potato
Drought tolerance Park et al. (2015)
AmDHN Ammopiptanthus mongolicus Alfalfa Cold tolerance Lizhen et al. (2015)
DREB1A Arabidopsis thaliana Tomato Cold tolerance Shah et al. (2015)
FvMYB1 Fraxinus velutina Tobacco Salt tolerance Li et al. (2016)
P5CS Vigna aconitifolia Kenyan cowpeas (Vigna unguiculata) Drought tolerance Okeyo-Ikawa et al. (2016)
2.5 Engineering for Molecular Farming/Pharming
Plants can be used to produce several chemicals and recombinant proteins that have strong effects on human health. In the light of this information, plant-manufactured chemicals are one of the most remarkable applications of transgenic plants. The cell's intrinsic metabolic processes are not designed for industrial production of these chemicals. Genetic engineering can manipulate metabolic pathways of cell, and resulted transgenic plants can synthesize more biopharmaceutical products and small chemicals suitable for industrial applications (Karuppusamy, 2009). There are many studies showing the successful production of specialty chemicals, biopharmaceuticals, and edible vaccines in seeds, leaves, or fruits (Hesselink et al., 2014; Niemer et al., 2014; Piller et al., 2005; Hudson et al., 2014; Lombardi et al., 2012; Kashani et al., 2012; Pniewski et al., 2011). In this context, especially the production of plant-based vaccines for human and animal was accepted as an attractive approach (Hudson et al., 2014). Up till now, several vaccine candidates with proved potential at the preclinical level were produced (Hernandez et al., 2014) Additionally, some of them are close to get license for implementation, such as a vaccine against influenza (Grabowski et al., 2014; Ward et al., 2014). In addition to the vaccines, other plant-based biopharmaceuticals are also approved for human usage. A good example for this application is Taliglucerase alfa, which is an enzyme produced in carrot cells after A. tumefaciens-mediated gene transfer. This plant-based chemical has already been approved and commercially available for the treatment of Gaucher's disease (Pastores et al., 2014). Although a variety of plant-based chemicals and vaccines have been produced, there are many challenges to overcome such as the timing of application, the vaccine, the dosage, and the capacity of the protein to induce immunity on oral administration (Yusibov et al., 2015).
Although there are many transgenic plants serving as biofactory for small chemicals and recombinant proteins obtained through A. tumefaciens-mediated gene transfer, A. rhizogenesinduced hairy root cultures are also good candidates to produce these substances. Different plant species especially medicinal ones have been transformed such as Callerya speciosa, Dracocephalum forrestii, and Artemisia tilesii (Yao et al., 2016; Weremczuk-Jeżyna et al., 2016; Matvieieva et al., 2016). Likewise, increased resveratrol content was obtained via A. rhizogenes-induced hairy root cultures of soybean (Kim et al., 2008).
3 VIRUS-INDUCED TRANSIENT GENE EXPRESSION IN PLANTS
Virus-induced gene silencing (VIGS) is mainly used for identification of gene function. This technique utilizes viral vectors that carry gene fragment of the target gene. As a result of virus induction, dsRNA molecule is produced, and this leads to starting of RNA-mediated gene silencing. VIGS is considered as a reverse genetic tool that provides an alternative way for characterization of gene functions in a transient way. In this part, mechanisms, development, and improvement of this method have been examined.
3.1 Basic Mechanism of VIGS
VIGS technique actually uses antiviral defense mechanism of plants in which posttranscriptional gene silencing (PTGS) occurs (Baulcombe, 1999a). In normal conditions, when
plants are infected with unmodified viruses, viral genome is targeted and destroyed. If the virus vectors carry a part of gene of interest, the corresponding mRNA of targeted gene is cleavage (Lu et al., 2003). VIGS term was firstly used by A. van Kammen who indicated resistance against viral infection in plants (van Kammen, 1997). Basically, this technique is based on silencing of gene of interest (GOI) through RNA-mediated defense system in plants. The main idea of VIGS includes transferring of viral RNA or DNA that also involves a small portion of specific gene sequence into plants (Baulcombe, 1999b). The target gene sequences are firstly inserted into viral genome without any disruption in its infectivity (Lu et al., 2003). So, this method carries an advantage that enables knocking out of a specific gene without affecting other genes in plant genome (Unver and Budak, 2009). In VIGS method, RNA-induced gene silencing mechanism occurs in which 21–25 nucleotide sequences of small interfering RNAs (siRNAs) are produced and directed to specific regions of target mRNAs for cleavage. This process takes place at posttranscriptional level (Fire et al., 1998; Klahre et al., 2002). From the long double-stranded RNAs (dsRNA), siRNAs are processed with RNAse-like enzyme known as DICER. At the end, siRNAs are connected to RNA-induced silencing complex known as a RISC (Unver and Budak, 2009). RISC containing siRNA has an ability to bind target mRNA region in which complementary with the specific siRNA is found. So, sense strand from target gene mRNA and antisense strand from siRNA are combined with each other, and specific regions of mRNA targets are degraded (Fig. 2) (Bartel, 2004).
This is the general mechanism for siRNA degradation process. Although all main steps are similar with siRNA degradation process, there are some details for VIGS method in plants for silencing of GOI. After the modification of viral genome that is joined with target gene portion, this construct is transformed into plants using A. tumefaciens. In the plant cell, foreign RNA molecule is transcribed and replicated by an endogenous RNA-dependent RNA polymerase (RDRP) enzyme. As a result, long dsRNA molecule is produced and then recognized by DICER. After the cleave of dsRNA into siRNAs, they are noted by RISC complex and converted them to single-stranded siRNAs. The RISC complex utilizes these single-stranded siRNAs to find out their complementary sequences in RNA pool of the cell. After finding right sequences, they are degraded (Ding and Voinnet, 2007; Waterhouse and Fusaro, 2006). Finally, target siRNAs are propagated and transported to other parts of plants for systematic gene silencing.
3.2 Methodology Development for VIGS
There are different types of viruses that are well adopted as VIGS vectors for silencing of target gene in plants. Both RNA (tobacco mosaic virus, TMV (Kumagai et al., 1995); potato virus X, PVX (Faivre-Rampant et al., 2004); tobacco rattle virus, TRV (Liu et al., 2002a); barley stripe mosaic virus, BSMV (Holzberg et al., 2002); bean pod mottle virus, BPMV (Zhang and Ghabrial, 2006); pea early browning virus, PEBV (Constantin et al., 2004); satellite tobacco mosaic virus, STMV (Gosselé et al., 2002); poplar mosaic virus, PopMV (Naylor et al., 2005); brome mosaic virus, BMV (Ding et al., 2006); and tomato bushy stunt virus, TBSV (Hou and Qiu, 2003)) and DNA viruses (tobacco golden mosaic virus, TGMV (Peele et al., 2001); cabbage leaf curl virus, CaLCuV (Turnage et al., 2002); African cassava mosaic virus, ACMV (Fofana et al., 2004); and tomato yellow leaf curl China virus, TYLCV (Tao and Zhou, 2004)) are used for VIGS applications in different silencing host plants. Among them, Nicotiana benthamiana, N. tabacum,
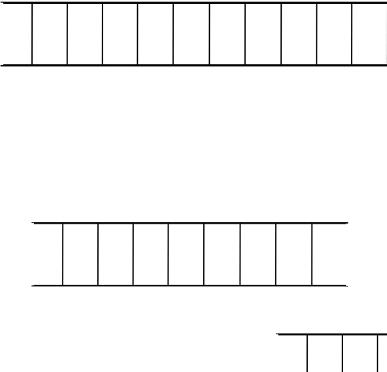
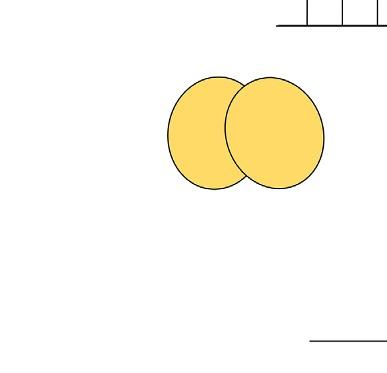
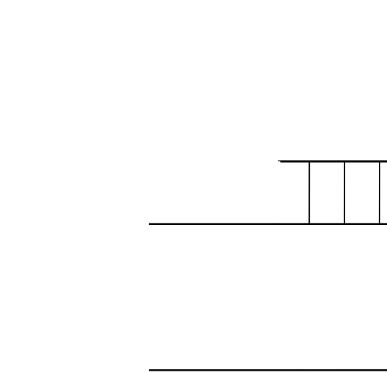
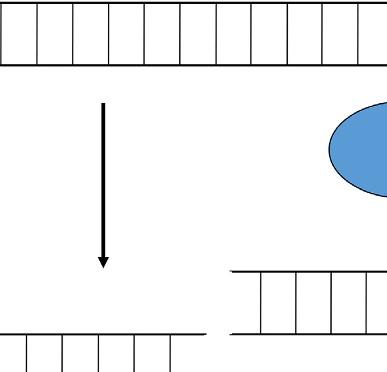
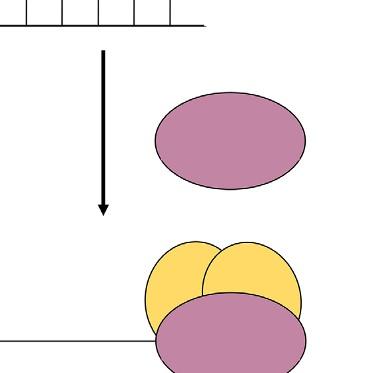
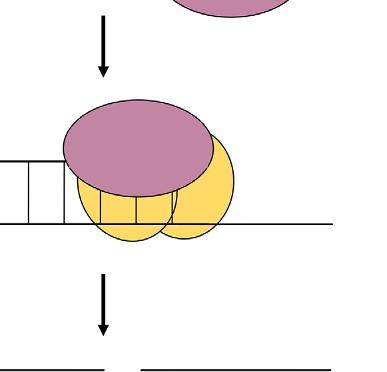
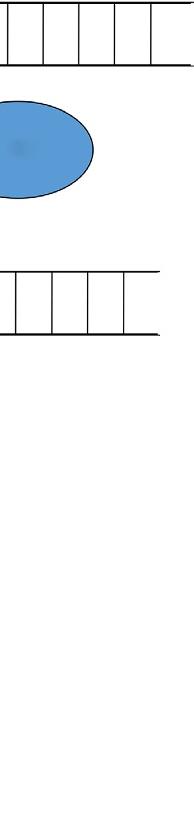
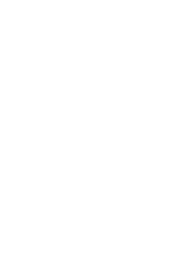
FIG. 2 Basic action mechanism of virus-induced gene silencing. VIGS, virus-induced gene silencing; RISC, RNA-induced silencing complex; and DICER, RNAse-like enzyme.
Solanum lycopersicum, A. thaliana, Capsicum annuum, Opium poppy, Aquilegia vulgaris, Hordeum vulgaris, Glycine max, Pisum sativum, Medicago truncatula, Lathyrus odoratus, Populus trichocarpa, Oryza sativa, Zea mays, Manihot esculenta, and Lycopersicon esculentum are widely used for host plant species in different gene silencing studies. Using VIGS method, there are many gene characterization studies such as different plant development stages (Senthil-Kumar et al., 2008), symbiosis (Grønlund et al., 2010), disease (van der Linde et al., 2011), nematode (Mao et al., 2011) and insect resistance (Mantelin et al., 2011), nutrient (Pacak et al., 2010), and abiotic stress (George et al., 2010).
The first VIGS application was performed with TMV that caused knockdown of pds gene in N. benthamiana (Kumagai et al., 1995). It was shown that the minimum sequence length of RNA for gene silencing was detected in different studies. They indicated that 23-nucleotide RNA was the minimum for 100% homology to the target gene. However, longer similar sequences were required for efficient PTGS (Thomas et al., 2001). Modified TRV is another VIGS vector that has been used for more than 15 years for gene silencing in plants. The main benefits of TRV vector are easy transfer into plants, especially Solanaceae family members and higher spreading capability throughout whole plant parts (Unver and Budak, 2009). Using this vector, gene silencing was succeeded in N. benthamiana (Liu et al., 2002a) and tomato (Liu et al., 2002b). Traditionally, VIGS vector is located between right and left borders of TDNA (Liu et al., 2002b; Feldman and Levy, 2012; Ratcliff et al., 2001). Strong promoters such as 35S or duplicate 35S promoters and terminator such as a ribozyme were added to cassette and inserted into A. tumefaciens. These regulators provide more effective and faster spreading of TRV vectors. pYL156 and pYL279 were TRV vectors with double 35S promoters that caused infection of different plant species (Liu et al., 2002a,b; Ratcliff et al., 2001). PVX is an RNA virus that has a limited host range when compared with TMV-based vectors. However, modified PVX vectors provide more stability than TMV vectors (Burch-Smith et al., 2004). So, we understand from the literature (Senthil-Kumar and Mysore, 2011a) that although VIGS technique has been performed for more than 20 years, there are several limitations and drawbacks for this gene silencing method. These problems have actually caused the development of some new methodologies to find out solutions. In this part, we summarized and listed some of the problems and their solutions:
(i) The main problem is to find right and suitable VIGS vectors for plant species. This can be solved by two ways. One is that, to silence gene in VIGS-recalcitrant species, heterologous gene sequences can be used from close relative of VIGS-compatible species (Senthil-Kumar et al., 2007; Zheng et al., 2011). The second is the development of new VIGS vectors with broad host range. So, an appropriate virus and vectors can infect several plant species (Senthil-Kumar et al., 2007).
(ii) Another drawback of VIGS method is the transfer of virus vector to plants. Generally, delivery of VIGS vectors to dicot plants is achieved by Agrobacterium , which is not suitable for monocots. Therefore, new strategies such as virus sap inoculation method ( Lu et al., 2003 ) or RNA transcript inoculation ( Ding et al., 2007 ) or DNA bombardment (Krenz et al., 2010) have been developed by different research groups.
(iii) There is an another problem arisen from movement of virus in plants that results in the lack of silencing in certain tissues ( Senthil-Kumar and Mysore, 2011a ). This can be achieved by keeping stable environmental conditions for proper virus movement ( Senthil-Kumar et al., 2008, 2007 ). In addition, suitable VIGS vectors should be selected for spreading of insert into plant tissues and should not possess a strong silencing suppressor. They should also carry a reporter gene whose expression provides visualization and discrimination of silenced tissues for interpretation of effect of silencing ( Senthil-Kumar and Mysore, 2011a ; Burch-Smith et al., 2004 ).
(iv) Both symptoms arisen from VIGS vector and virus cause a prevention of interpretation of data. VIGS vectors that manufacture serious signs in host plants should be evaded