ENGINEERING MEDICINE ENGINEERING MEDICINE ENGINEERING MEDICINE
THEPAST,PRESENT,ANDFUTUREOFHEALTHCAREINNOVATION
Biomedical Devices and R o scitob
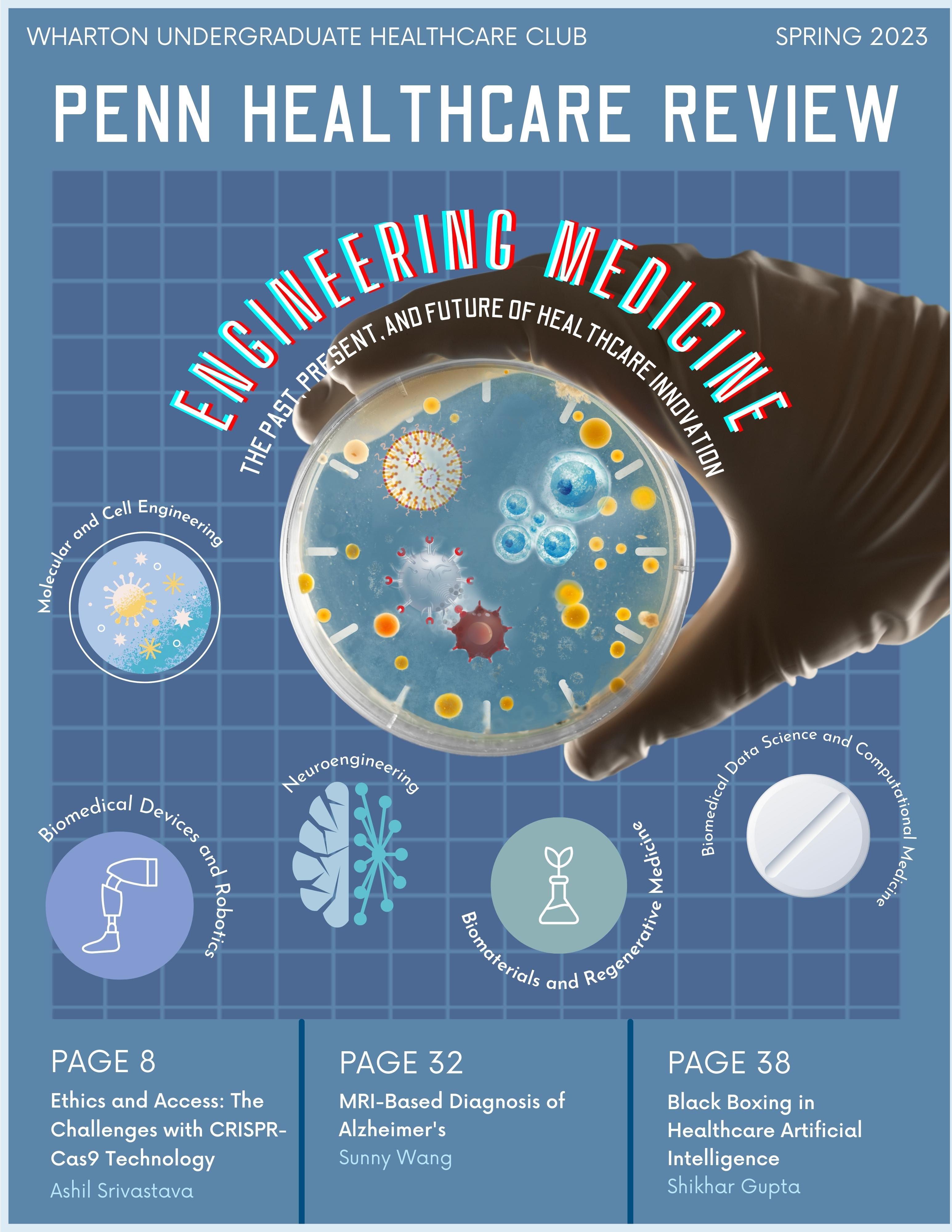
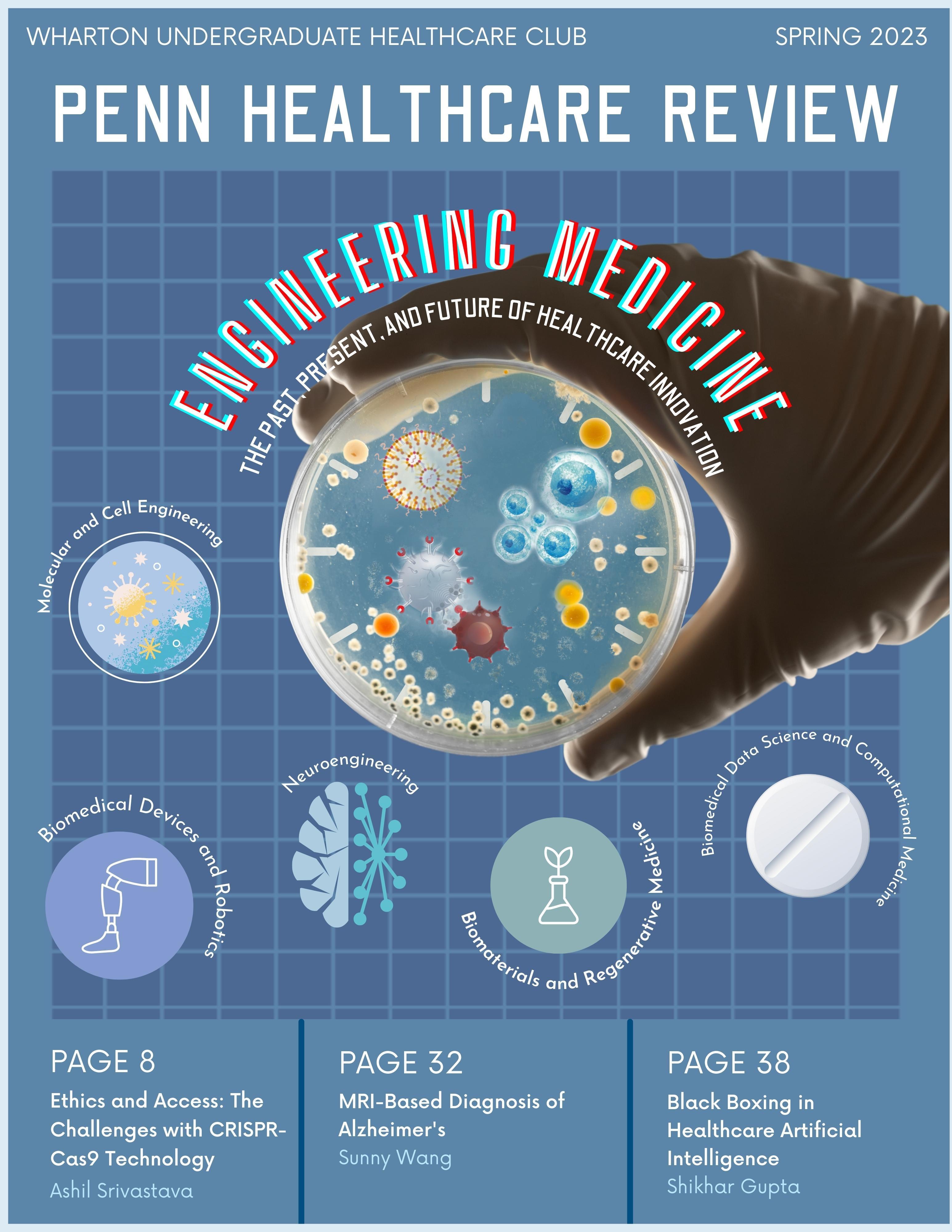
PAGE 11 PAGE 19
EthicsandAccess:The ChallengeswithCRISPRCas9Technology
AnnaShell
The Organ Crisis and How Artificial Organs Present a Solution
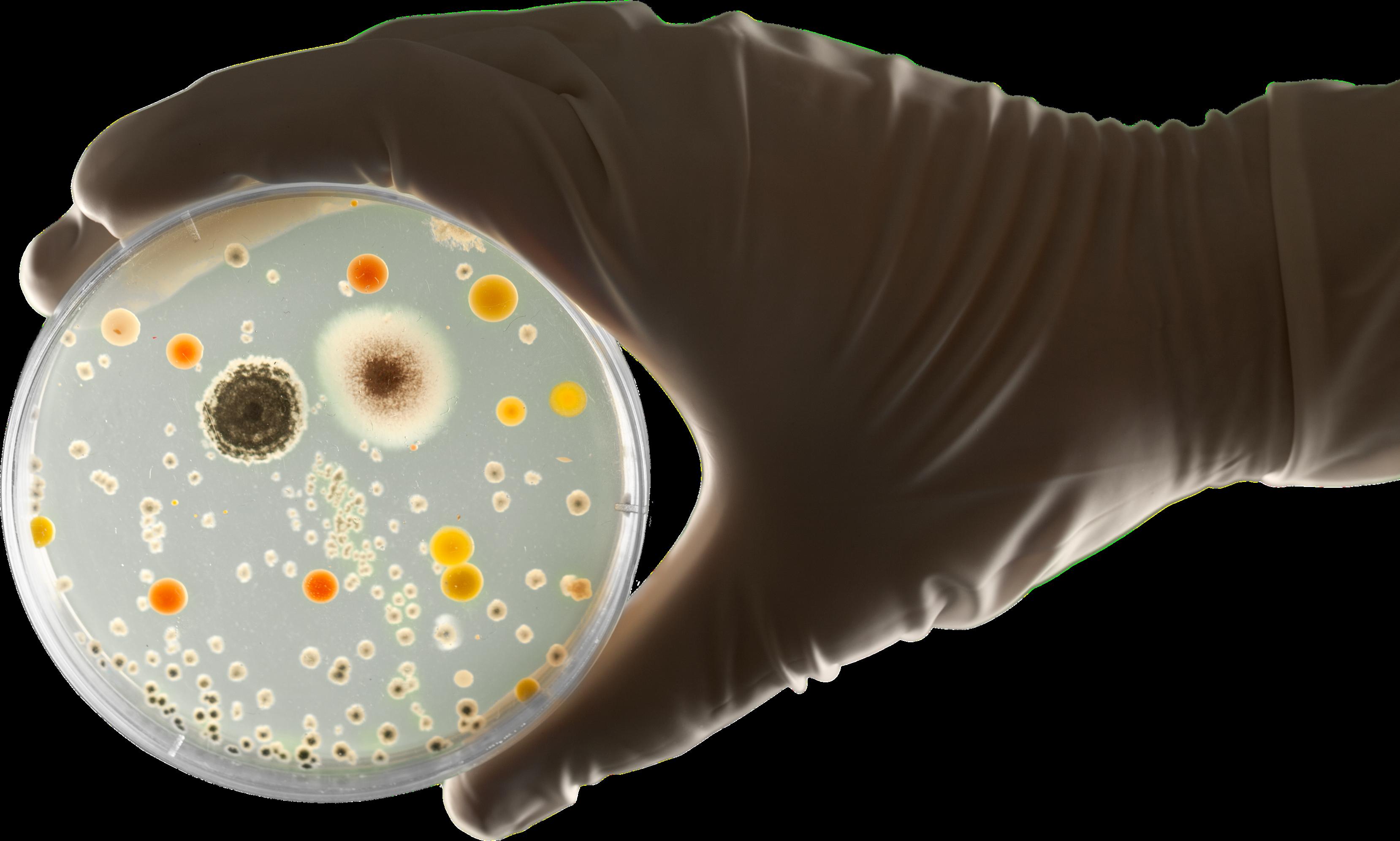
Nolan Anthony
dicalDataScience andComputati on a l enicideM
PAGE 41
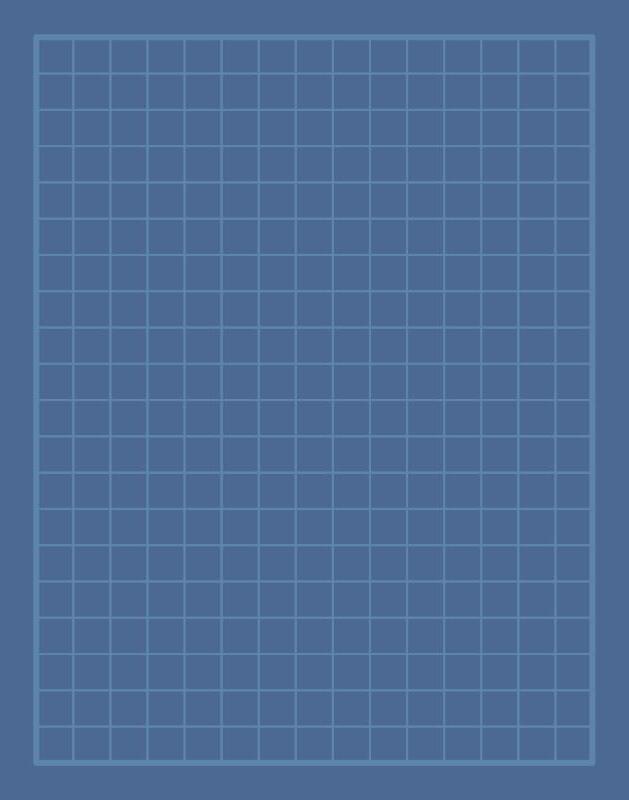
Black Boxing in Healthcare Artificial Intelligence
Shikhar Gupta
Dear Readers,
The landscape of medicine is changing rapidly We have now reached a point where progress in medicine is heavily reliant on the advancements of seemingly unrelated fields. Therefore, it’s increasingly important that future healthcare leaders are equipped with a strong understanding of how to leverage core insights from these different fields to push the frontiers of medicine, whether they be sociology or economics. Or even engineering.
This semester, I’m excited to share the deeply inspiring articles written, edited, and designed by our 60+ team members that explore the intersection between engineering and medicine. This is the largest and most diverse cohort of students in the history of the Penn Healthcare Review, and I’m excited to share their hard work and brilliance with the rest of the world.
These articles investigate topics ranging from lipid nanoparticles to neural mapping, leveraging primary research from interviews with thought-leaders like Dr Edward Stadtmauer from Penn Medicine and Dr. Roger Hartl from Weill Cornell Medicine. Students also conducted in-depth literature reviews, solicited feedback from professors, and iterated their work constantly using peer review.
In this edition, I’m also deeply honored to feature an interview with Dr. Katalin Karikó, one of the pioneers behind the non-immunogenic, nucleoside-modified RNA technology used in the COVID-19 vaccines. We discussed how this breakthrough technology was developed, other novel applications of mRNA, and advice for aspiring researchers looking to make their mark in the field of molecular and genetic engineering.
We hope that the Engineering Issue leaves you with a deeper understanding of the remarkable potential of these advancements in revolutionizing medicine
Enjoy!
Om Gandhi Editor in Chief, Penn Healthcare Review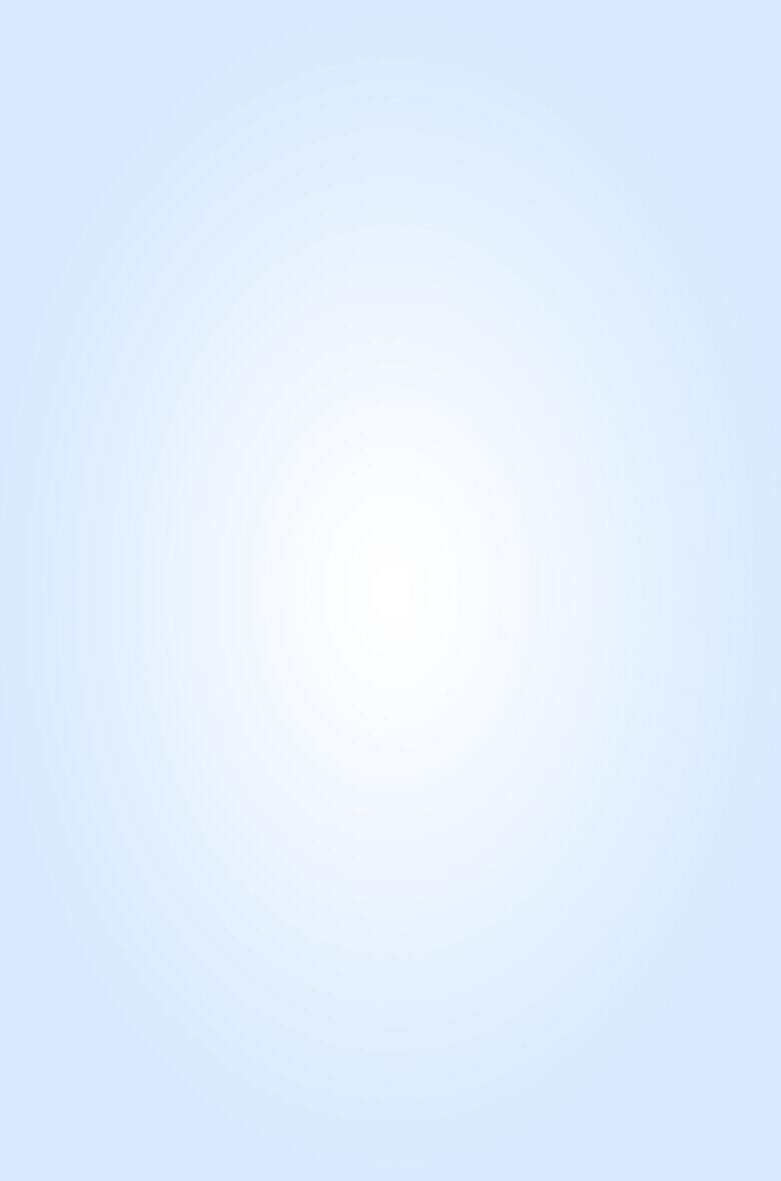
EDITOR-IN-CHIEF
Om Gandhi
HEAD OF DESIGN
Julia Gerbino
EXECUTIVE EDITORS
Tingting Chung
Jaskeerat Gujral
Arjan Kahlon
Adanna Mogbo
Aarsha Shah
WRITERS
Nolan Anthony
Shahana Banerjee
Tingting Chung
Alex Gerlach
Jaskeerat Gujral
Shikhar Gupta
Arjan Kahlon
Srijan Kalva
Eric Lee
Ryo Lindsey
Grace Matwijec
Wendell OderkirkAlvidrez
Tereza Okalova
Manav Parikh
Kayla Patel
Anna Shell
Saraswati Sridhar
Ashil Srivastava
Neil Tangal
Sunny Wang
EDITORS
Ola Adio
Eesha Balar
Shahana Banerjee
Jerry Cai
Ashrit Challa
Siddharth Chitta
Ella Eseigbe
Michael Go
Anagha Gouru
Jessica Guo
Shikhar Gupta
Hiba Jamil
Srijan Kalva
Andrew Lee
Arav Nangia
Maya Narang
Manav Parikh
Austin Pothikamjorn
Luna Sato
Aarsha Shah
Saraswati Sridhar
Shaira Tabassum
Vicky Vo
Rebecca Wang
Jessica Wu
Vivian Yao
Megan Zhang
DESIGNERS
Oscar Capraro
Siri Dandu
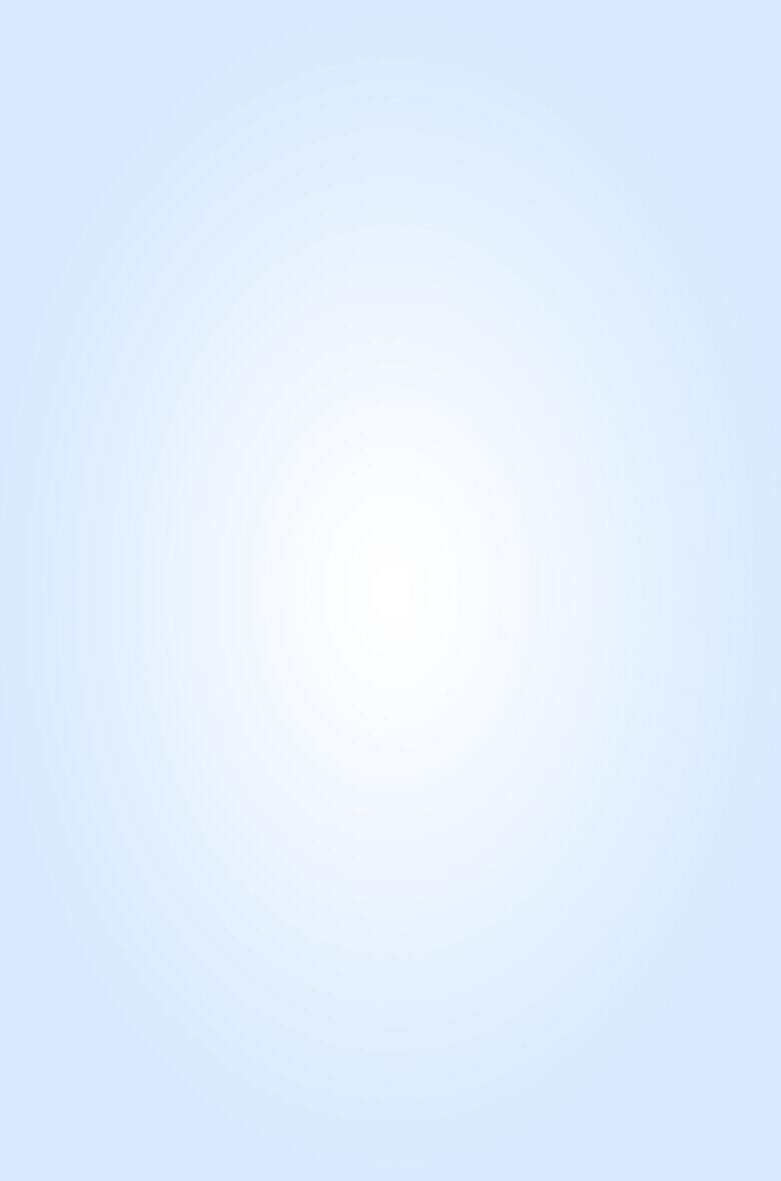
Julia Gerbino
Laura Jannetta
Nancy Lam
Jenny Li
Cherry Lin
Erin Ma
Josh Mukherjee
Janine Navalta
Zachary Rentala
Antonia Solar
Ashley To
11
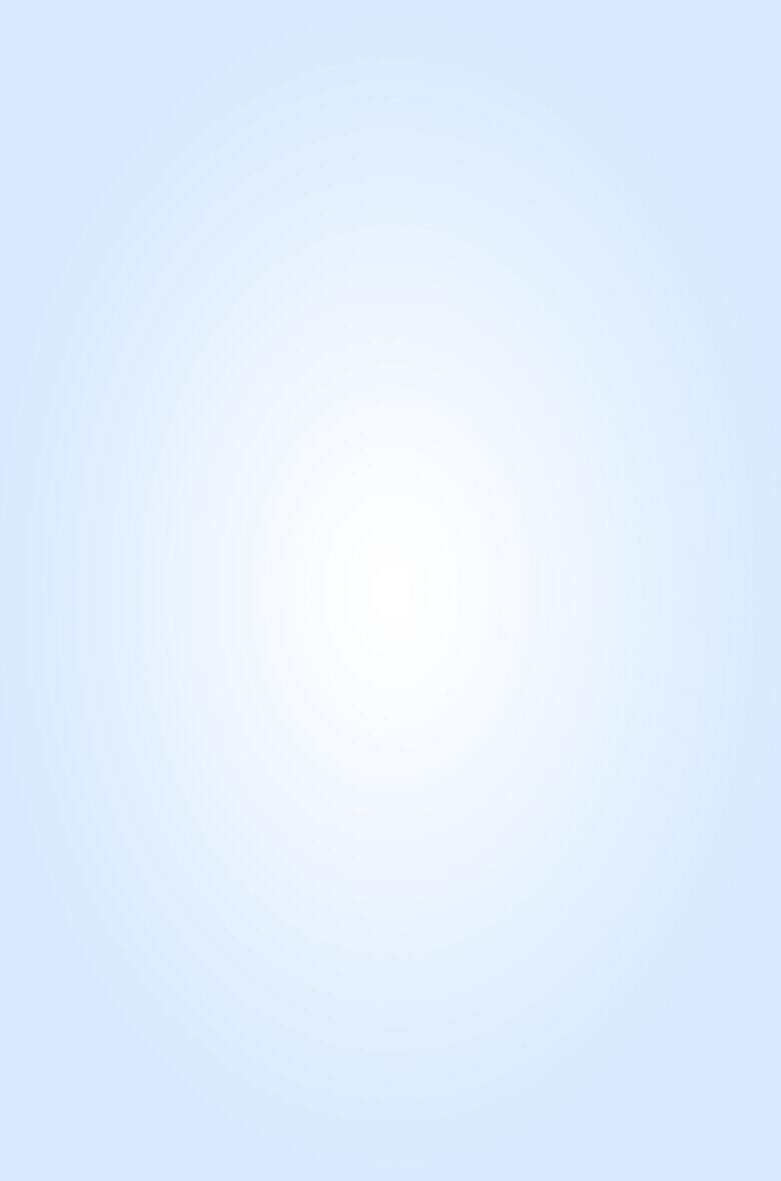
MOLECULAR AND CELL ENGINEERING
Nanomedicine: An Emerging Frontier in Cancer Therapeutics
Written by Grace Matwijec, Edited by Eesha Balar, Designed by Jenny Li
Lipid Nanoparticles as a Novel Strategy to Traverse the Blood-Brain Barrier in Glioblastoma Patients
Written by Saraswati Sridhar, Edited by Maya Narang, Designed by Siri Dandu
Ethics and Access: The Challenges with CRISPR-Cas9 Technology
Written by Anna Shell, Edited by Saraswati Sridhar, Designed by Jenny Li
CARs Drive Innovation in Deadly Diseases
Written by Eric Lee, Edited by Aarsha Shah, Designed by Siri Dandu
Revolutionizing Vaccines: The Development and Significance of mRNA Technology
Written by Jaskeerat Gujral, Edited by Jerry Cai, Designed by Laura Jannetta
BIOMATERIALS AND REGENERATIVE MEDICINE
The Biomechanics of Synthesized Tissue: A Study of Stem Cells in Tissue Engineering
Written by Arjan Kahlon, Edited by Megan Zhang, Designed by Janine Navalta
The Organ Crisis and How Artificial Organs Present a Solution
Written by Nolan Anthony, Edited by Arjan Kahlon, Designed by Janine Navalta
Exciting Ways of Reducing Excitation: Stem Cell Therapy and its Antiepileptic Potential
Written by Tereza Okalova, Edited by Ella Eseigbe, Designed by Cherry Lin
Stem Cell Therapeutics for Traumatic Brain Injuries
Written by Srijan Kalva, Edited by Arjan Kahlon, Designed by Cherry Lin
BIOMEDICAL DEVICES AND
ROBOTICS
BrainLab: Advancements in Robotic Neurosurgery
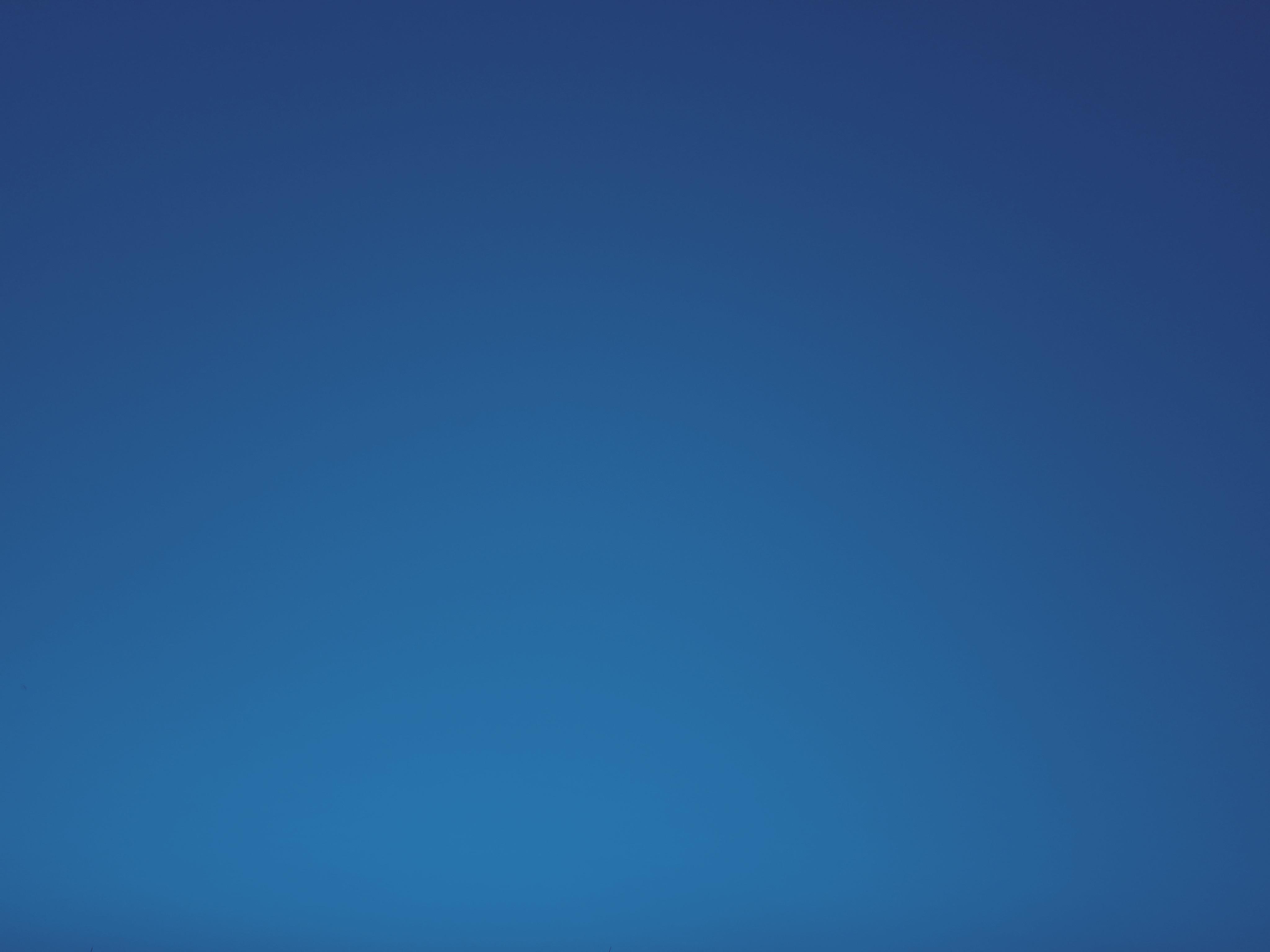
Written by Jaskeerat Gujral, Edited by Austin Pothikamjorn, Designed by Josh Mukherjee
Revolutionizing Medical Surgery with Steerable Needles
Written by Ryo Lindsey, Edited by Andrew Lee, Designed by Josh Mukherjee
Leadless Pacemakers: Setting the Pace for the Future of Electrophysiology
Written by Ashil Srivastava, Edited by Jessica Wu, Designed by Julia Gerbino
Organ-on-a-chip: The Future of Biomedical Research
Written by Alex Gerlach, Edited by Ashrit Challa, Designed by Ashley To
NEUROENGINEERING
Stereoelectroencephalography: A Breakthrough in Mapping Neu
Written by Tingting Chung, Edited by Vicky
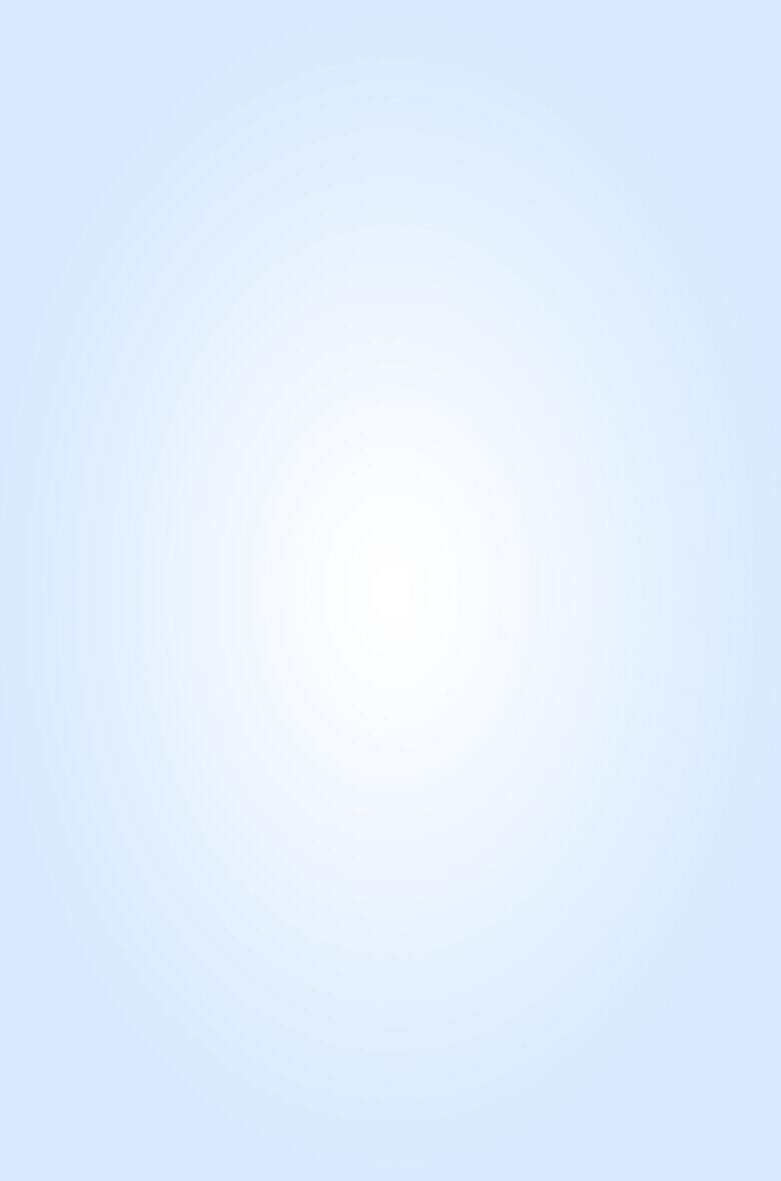
Alzheimer’s Disease Diagnosis U
Written by Sunny Wang, Edited by Jessica G
To Be Or Not To Be? The Curiou
Written by Neil Tangal, Edited by Hiba Jamil The
BIOMEDICAL DATA SCIENCE AND COMPUTATIONAL MEDICINE
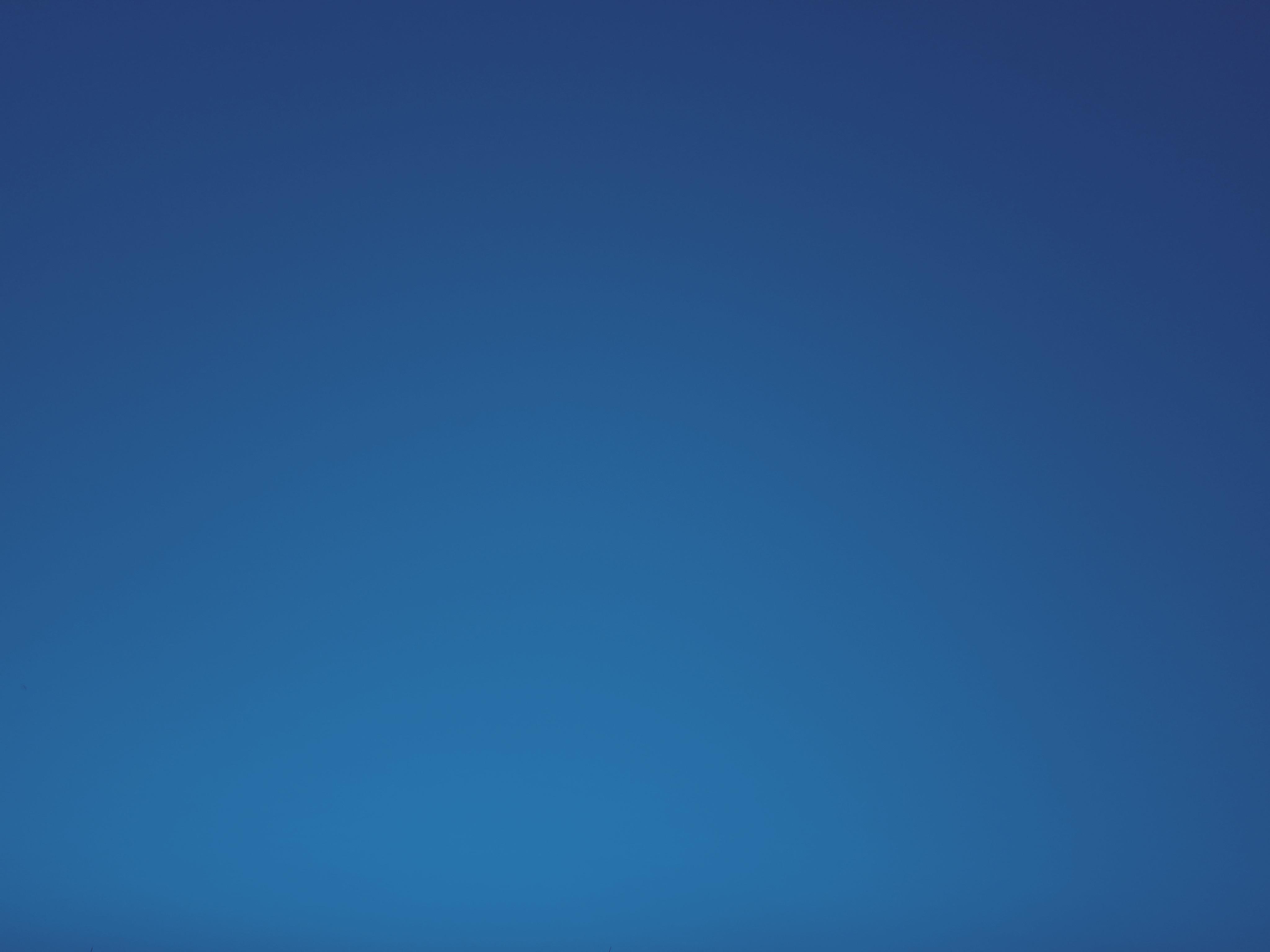
Written by Shikhar Gupta, Edited by Vivian Yao, Designed by Erin Ma
Written by Shahana Banerjee, Edited by Adanna Mogbo and Manav Parikh, Designed by Antonia Solar
Leveraging AI in Radiomics
Written by Kayla Patel, Edited by Shikhar Gupta, Shahana Banerjee, and Anagha Gouru, Designed by Erin Ma
Dr. Katalin Karikó
Katalin Karikó, PhD, is a biochemist and researcher, best known for her contributions to mRNA technology and the COVID-19 vaccines. Dr. Karikó and co-collaborator Drew Weissman, MD, PhD, invented the modified mRNA technology used in Pfizer-BioNTech and Moderna’s vaccines to prevent COVID-19 infection. Dr. Karikó is a senior vice president at BioNTech and an adjunct professor of Neurosurgery at the University of Pennsylvania. She joined the Perelman School of Medicine at the University of Pennsylvania in 1989 and began collaborating with Dr Weissman in 1997 Dr Karikó received her bachelor's degree in biology in 1978 and her doctorate in biochemistry in 1982 from the University of Szeged in her native Hungary.
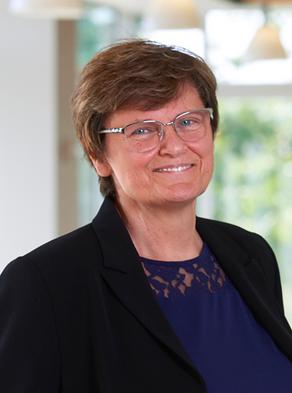
Can you tell us more about your pioneering work in the field of mRNA research?
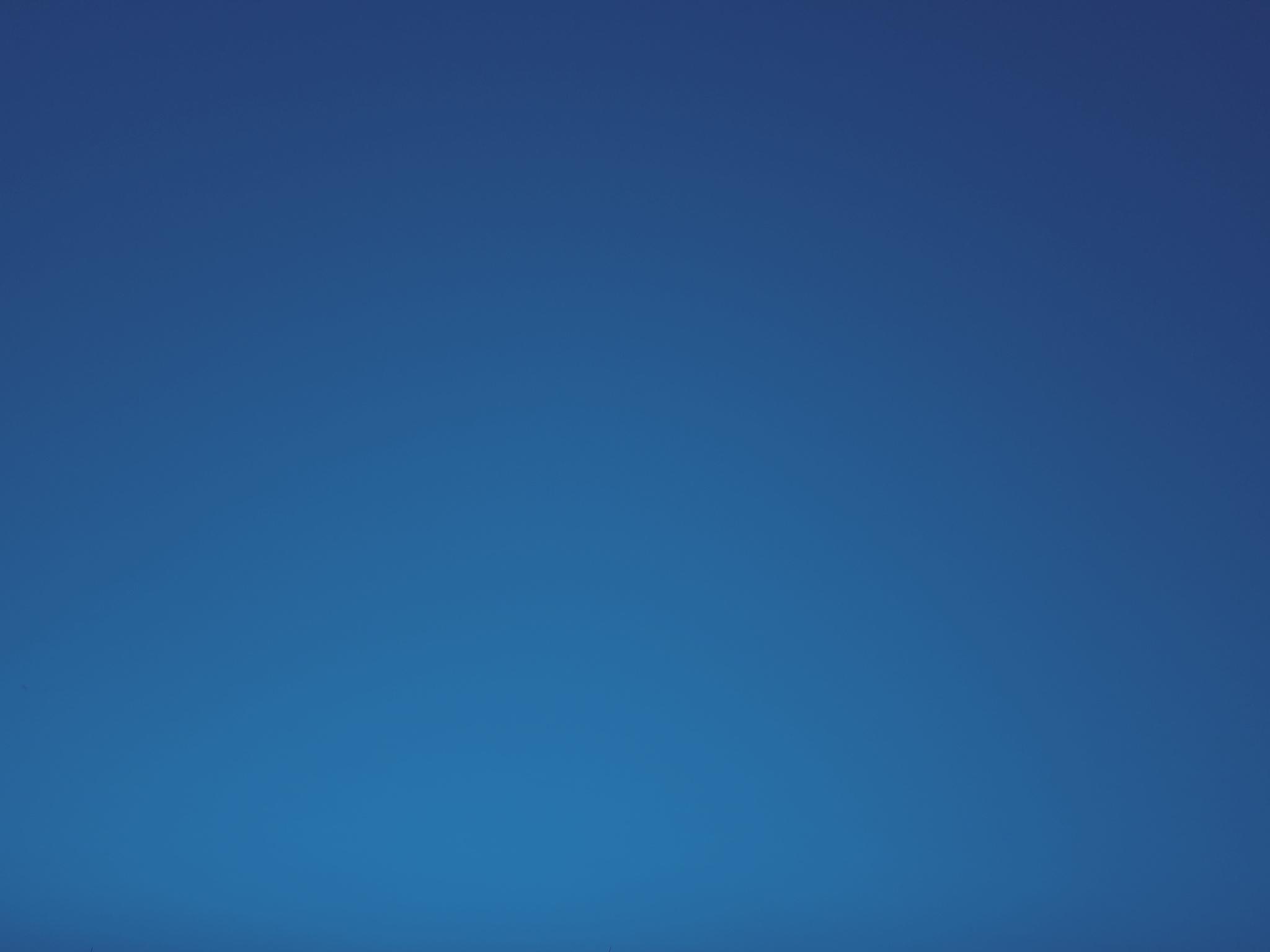
I was working with RNA in Hungary and then started to work at Penn in 1989 I wanted to develop RNA therapy to treat conditions. My initial work revolved around cardiology, and then I transitioned into neurosurgery research for 17 years. It is interesting how you can make innovations by combining knowledge from various fields For instance, an article will be released by Harvard Business Review that discusses how there was an expert in cardiology and a physician that knew how to reach certain blood vessels in the brain; the duo is able to combine their unique talents.
My own focus was on developing nonimmunogenic RNA therapy, and after a decade of research, I discovered that RNA itself was nonimmunogenic With each new enzyme that became available, I continued to improve the RNA, which was critical to the success of the project. Seeing improvement over time was highly motivating and drove me to continue refining the therapy.
What motivated you to focus on mRNA as a potential therapeutic tool?
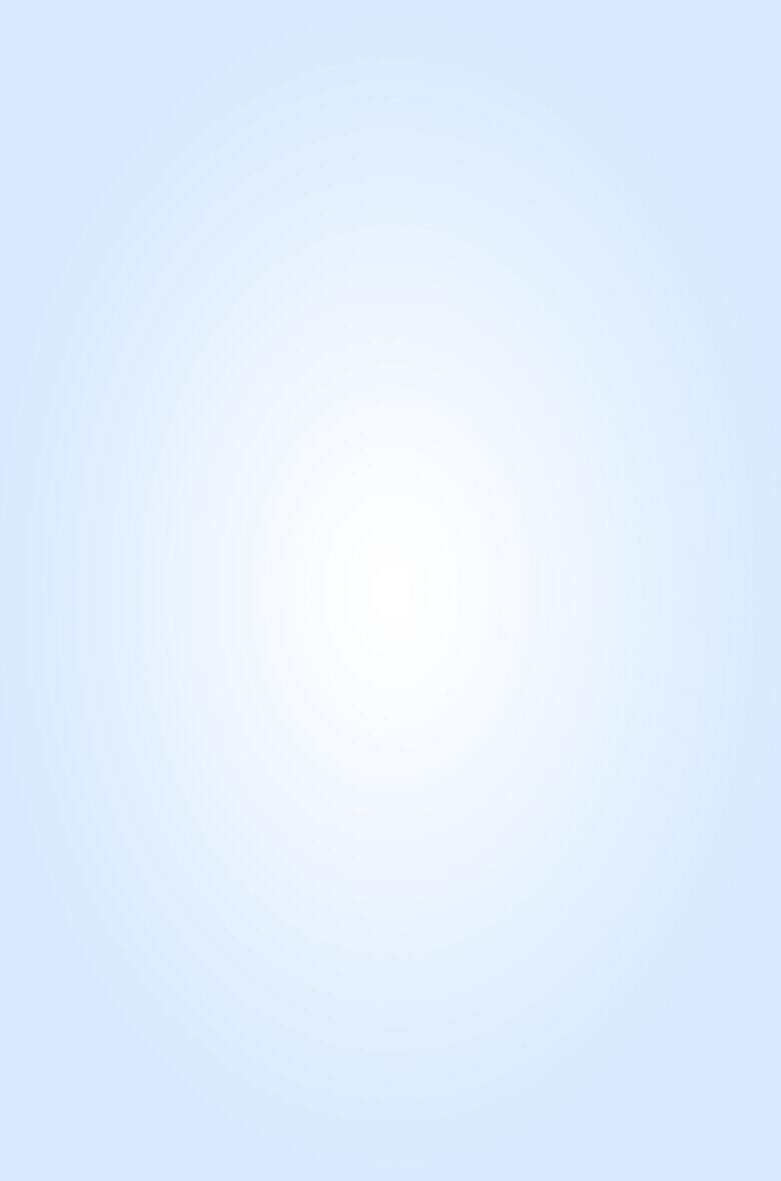
I was collecting knowledge in the field of RNA. Here in Hungary, I was synthesizing, those were short molecules.
I initially worked at Temple University and did a Human trial for HIV patients; we also used double-stranded RNA. Everything that I did involved RNA; we conducted a clinical trial in 1986 with double-stranded RNA What kept my interest was that I could see improvement. It is critical to see progress to pursue something.
Many people received grants, money, and promotions. I did not have any of these things, but I could see that the project was improving. I am trying to emphasize here that you are young and should be enthusiastic about certain diseases or certain things to focus on Many people used to focus on research. Now, it is backward, and the goal is to get chairmanship or money. That's the kind of thing that drives nowadays. There are fake papers because that's how you get positions in certain institutions. People want awards and positions
I never wanted the recognition; for me, it was enough that I knew what I was doing. I was not craving the attention Anyway, it was not important for me to get promotions. I got demoted and never got NIH R01 grant. We saw a biological function of the protein that could have a physiological difference.
The next step was to see humans, but I never expected it to show. I was kicked out of Penn they showed me the door. Another lesson that I can give is don't let others define you, or think you are useless. I would have not been able to achieve any of this. It is important to think about what is next. Don't spend time licking your wounds and feeling bad for yourself.
What other potential applications do you think we can explore with mRNA technology?
In 2018, mRNA was used to treat a patient with heart failure, which was in a phase 2 trial. The trial was very successful The mRNA was injected into the patient, which coded to make new blood vessels. That was known to be a therapeutic method. Also, mRNA is used for gene editing and interrupting genes responsible for amyloidosis to treat the production of toxic proteins 1 5 years later the patient did not make toxic proteins. You can see that mRNA was applied to other conditions, not just viral vaccines, bacteria, malaria, and parasites, that are not human trials. The applications of mRNA in influenza and HIV are in clinical trials. Of course, many companies are specializing in certain applications and fields.
We still advise my team and remain as a consultant. We are working on different applications of mRNA I have other things besides mRNA that I would like to focus on. I have to attend ceremonies in Berlin. I don't like awards, I think research is very complex, and you pick one person. I think many people contributed to the work throughout the years, and it is unfair For 40 years, I did not get anything, and I was ok with that. Then, in 1.5 years, I got 100 awards.
Can you provide any advice for young researchers looking to make their own contributions to the field of mRNA research, or genetic engineering as a whole?
The most important thing is that they have to like what they are doing They should not be concerned about chasing the money. As I mentioned, they can go to a team that is already working in the field and learn and move on and meet other colleagues in different fields educating each other. That's what we did with Drew Weissman He didn't know about RNA and mRNA therapy work, and I did not know immunology. I was learning how to do immunology, and he was learning RNA, and we were both figuring out what we can do.
What are you currently working on, more than a decade after your initial work in the field?
Currently, I am working at BioNTech. For 9 years, I was commuting between Frankfurt and Philadelphia.
It is important to associate with others It is important to be nice to everyone. They should not get intimidated because the knowledge is tremendous. I suggest you do something; it is important to shadow and learn. Of course, it is good to know a lot, but sometimes it is also good when you don’t know things because you can get experience. It is important to learn the basic experiments. You should pick something that you would want to spend all your life on to solve the problem.
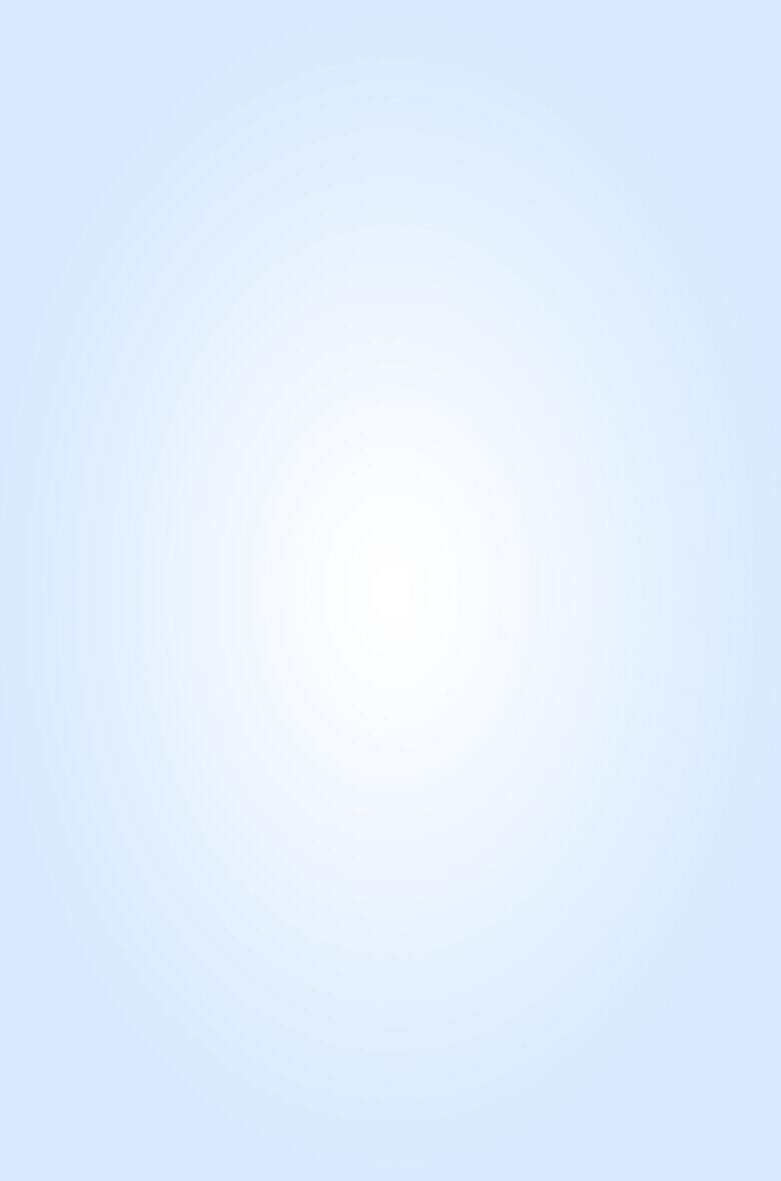
NANOMEDICINE An Emerging Frontier in Cancer Therapeutics
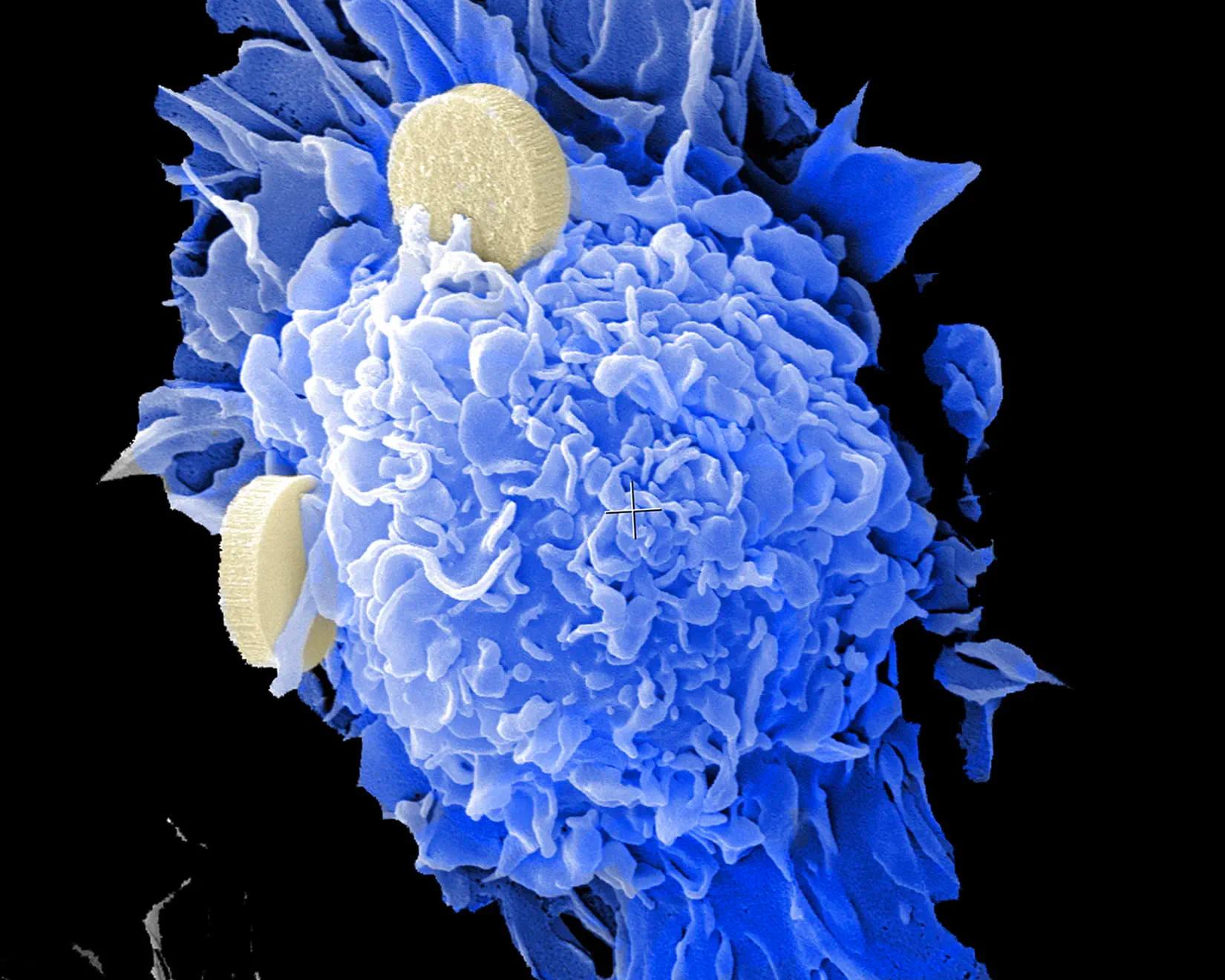
Introduction
Nanomaterials have revolutionized various fields, such as the agricultural industry, textile industry, and most recently the medical industry.
Nanotechnology utilizes atomic and molecular techniques to make particles that are nano-scale (one-billionth) in size. Since biological machinery and systems within the cell operate at the nanoscale, nanomaterials can improve the way synthetic materials interact with biological systems, thus improving therapeutics Nanomaterials made from inorganic or organic materials have a variety of clinical applications and have been applied in a variety of areas such as regenerative medicine and molecular level diagnostics, with their newest application being in oncology The medical community has begun to utilize nanomaterials in the clinical setting for cancer patients and still continue to explore nanomaterials’ potential applications today as the newest frontier in cancer therapeutics
Nanomaterial-Based Drug-Delivery Systems
Due to nanoparticles’ ability to provide targeted delivery of and controlled release of drugs, a drug delivery system (DDS) using nanoparticles as “carriers” is more advantageous for cancer patients. Scientists in nanomedicine have the capability to design custom nanoparticles specific to a target cell environment, such as adding a ligand on the surface of a nanoparticle, so they can bind with a
specific cell-surface receptor [1] Therefore, by attaching drugs to nanoparticles with features designed for binding to a specific target cell population, drugs can be delivered to specific cells, making drug delivery more effective and locally applied (see Figure1) This mitigates damage to surrounding tissues while also increasing the accumulation of a drug at its target site, resulting in a lower dose needed to receive the same result in comparison to traditional non-specific drug delivery [2]. This is critical for cancer patients given that damage to surrounding tissues from treatment and drug toxicity still remain large obstacles in cancer treatment [3].
Research has shown that nanotechnology-based DDS provides an alternative method of delivering cancer drugs that effectively mitigates both these problems. For instance, an in vitro study from 2006 demonstrated that nanoparticles could effectively kill cancer cells without harming surrounding tissues [5] Researchers attached anti-HER-2 antibodies to the nanoshells to bind to the HER-2 receptors, which are part of a larger signaling cascade, on the surface of the breast cancer cells These nanoshells then caused cancer cell death through photoablation without harming healthy cells, since the nanoshells were only bound to the cancerous cells [6] Another study from 2010 demonstrated the role that nanoparticles can play in increased dosage without increased toxicity A phase I dose escalation clinical trial involving a nanoparticle-based DDS attached chemotherapy drugs to the surface of 27-nm gold
particles, which allowed a higher dose of these drugs to be administered above the toxicity threshold since the drug administration was localized to the target area [7] Due to years of research, now these nanomaterial-based DDS are largely entering the clinical setting A handful of nanoparticle-based DDS are on the market, such as Doxil® and Abraxane®, while many others are still in clinical trials [8]
Nanomaterial T-Cell Based Therapy
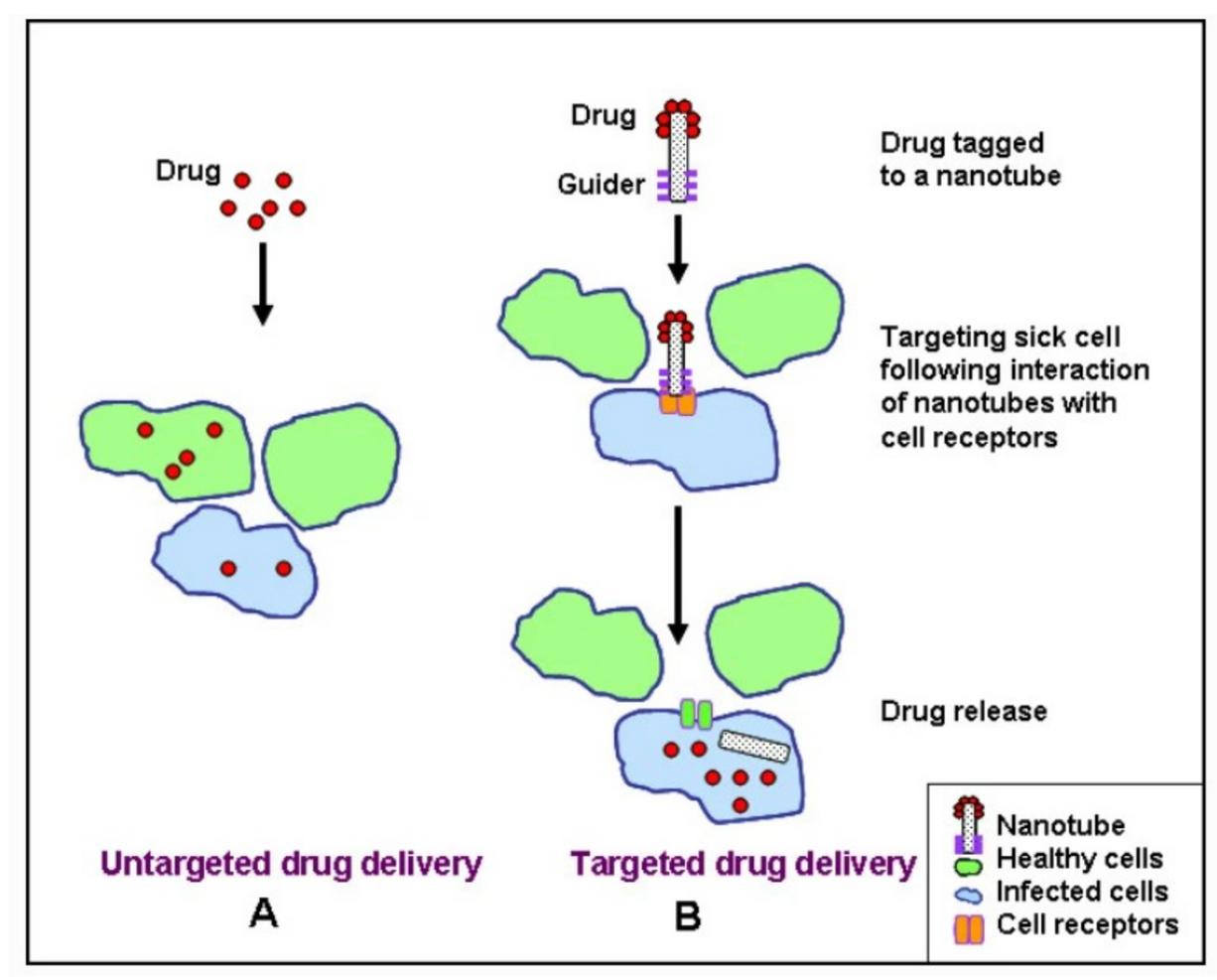
T-cell based immunotherapy is another promising treatment for various cancers; however, various barriers can hinder T-cells from effectively targeting cancer cells. Recently, researchers have turned to nanomaterials as a potential solution to resolve these barriers. One such challenge of in-vivo T-cell therapy has been effective enhancement of ex-vivo T-cells once injected into the body, meaning having a high enough number of manufactured T-cells to effectively target cancer cells [9] Nanoparticles pose a unique solution to this problem because they can be designed to stimulate in-vivo T-cell growth by designing them with stimulatory cues to produce in-vivo T-cells Therefore, this would eliminate the need for ex-vivo manufactured T-cells injected into the body all together Another challenge of T-cell based therapies is often effective penetration of Tcells into cancer cells [10] With nanomaterials, penetration could be enhanced since nanomaterials can be engineered to target inhibitive and physical barriers on cancer cells that typically inhibit T-cells Therefore, application of nanomaterials could drastically improve promising T-cell therapies for cancer patients in the near future.
Drawbacks
There are still multiple areas of concern about the toxicological consequences of engineered nanomaterials in the body, despite increasing usage of nanomedicine. One such area of concern is the potential for nanoparticles to penetrate cell membranes of unintended areas in the body, such as the blood-brain barrier Due to the properties of nanoparticles, it is difficult for researchers to predict the biodistribution of these particles once in the body and for how long they will stay in a particular organ Particularly, nanoparticles that are composed of inorganic compounds, which cannot be digested and broken down, can accumulate in the body and potentially aggregate, causing unknown effects Moreover, given the high surface area-to-volume ratio of nanoparticles, they are highly reactive, which could prove toxic to the body For instance, nanoparticles, which can easily penetrate cell membranes, could bind with a toxin, which otherwise would not have been able to enter the cell [11].
Despite these risks, many in the scientific community believe that the dramatic reduction in toxicity the nanoparticle DDS provides for cancer patients outweighs any potential toxicity from use of the nanoparticles. Some also believe that such toxicity concerns for nanomedicines are exaggerated given that nanomaterials have been used in the food sector industry for years and no reports of any toxicity associated with nanomaterials in food have been registered [12]
Conclusion
Nanomedicine is an exciting frontier for cancer researchers to continue creating better therapeutics. As of now, the most developed applications of this technology in cancer medicine are nanoparticle-based drug delivery systems, which result in more effective and localized drug delivery, and newer applications of this technology are being investigated, such as nanoparticle T-cell based therapies, which could eradicate current challenges faced with T-cell based therapies While there are some concerns about the unintended effects nanoparticles could have on the body, nanotechnology applications in cancer therapeutics are still very promising and may improve current treatment options and ultimately patient outcomes
LIPID NANOPARTICLES
As a Novel Strategy to Traverse the Blood-Brain Barrier in Glioblastoma Patients
Written by: Saraswati Sridhar Edited by: Maya Narang Designed by: Siri DanduGlioblastoma (GBM), also known as a grade IV astrocytoma, refers to an aggressive brain tumor that spreads rapidly into the deepest tissues of the human brain, typically the frontal or temporal lobes It can arise on its own or from a lower-grade astrocytoma, which typically forms in the brain and spinal cord Symptoms often include persistent headaches, blurred or double vision, vomiting, loss of appetite, cognitive and mood changes, new seizure onset, and gradual onset of speech difficulty GBM is the most common brain and Central Nervous System (CNS) tumor, accounting for almost 50% of all cases with a typical prognosis of about 12-18 months and a five-year survival rate of less than 10% [1]
GBM remains one of the most difficult tumors to treat with complex regimens of radiation, surgery to safely remove as much of the tumor as possible, and chemotherapy (primarily temozolomide) for symptom mitigation Numerous factors contribute to the challenges of treating GBM, including the blockage of drug entry into the CNS by endothelial cells in the blood-brain barrier, high genetic heterogeneity, high migratory capacity, and rapid and pervasive tumor growth [2] This barrier allows only select molecules to pass from the bloodstream to pass into the cerebrospinal fluid from the bloodstream, preventing the entrance of both small-molecule and macromolecule drugs into the brain [4]
Direct administration of drugs to the CNS is invasive, has a high risk of brain damage and infections, and has limited assimilation [3] Blockage of drug entry by the blood-brain barrier further complicates the process of delivering enough drug to kill tumor cells, but not enough to produce cytotoxicity
One solution addressing these treatment barriers involves the use of lipid-nanoparticles to deliver chemotherapeutics past this barrier and into the brain, while targeting malignant tumor cells and sparing healthy neurons Lipid nanoparticles (LNPs), one of the most widely-studied nanomaterials, are spherical particles composed of ionizable lipids LNPs have demonstrated tremendous promise in clinical trials for site-specific drug delivery for a variety of therapeutic agents, primarily due to their biocompatibility, biodegradability, and entrapment efficiency [5] The attractiveness of utilizing LNPs to target tumor cells in the brain is a result of their physicochemical properties, such as their polar surface area They typically enter the cell through endocytosis and increase in quantity once inside the cell; tumor cells often have a high capacity for LNP uptake resulting from the presence of target cell surface ligands [6].
While many techniques and applications of LNPs in GBM treatment are under development, the two primary approaches comprise direct delivery In one such approach, the drug undergoes complexation with a polymer and is administered to the patient in the form of a polymeric nanoparticle Another approach is cell-based delivery, where a nanoparticle with a therapeutic transgene is incorporated into stem cells, which are then multiplied in vivo and readministered to the patient Mesenchymal stem cells are especially promising for the latter application, as they can efficiently target tumor cells by bypassing the blood-brain barrier and traveling large distances [7] LNPs are typically synthesized through layerby-layer assembly, a technique developed by the Hammond laboratory at MIT which results in surface-functionalized nanoparticles that carry
drugs in their core These nanoparticles, when coated with the AP2 peptide, which mediates clathrin-driven endocytosis, come into contact with the LRP1 receptor This receptor, frequently found in tumors in a mutated form, normally acts as a tumor suppressor As a result, these nanoparticles are most effective at penetrating the vessels that form the blood-brain barrier, without inflicting damage on non-tumor cells [8] The effectiveness and precision of LNPs can also be augmented using carbonized quantum dots to form Large Amino Acid-Mimicking Carbon Quantum Dots (LAAM-CQDs), which are photoluminescent nanoparticles that can be engineered to mimic the structure of large amino acids and carry aromatic drugs for delivery to the specified tumor sites These dots often display high tumor selectivity and are highly useful for imaging and diagnostics as well [9]
One treatment modality that is often carried out through drug delivery via LNPs is RNA interference (RNAi), where the introduction of short interfering RNA (siRNA) suppresses gene expression with a high level of specificity, and can be used to eliminate the expression of genes that promote cancer cell survival, formation, and migration [10] A recent development significantly facilitating RNAi in tumor cells is the development of poly(beta-amino ester) or PBAE, which forms electrostatic complexes with negatively-charged cargo-like nucleic acids This polymer is degradable by both hydrolysis and bioreduction, leading to low cytotoxicity As a result, the use of PBAE nanoparticles have shown effectiveness in both gene knockdown and reduction in cancer cell viability [11]
LNPs can also be derived from neurotransmitters, in the form of neurotransmitter-based lipidoids (NT-based lipidoids), which are synthesized using chemicals naturally occurring in the brain (neurotransmitters) as a base. Some NTs, such as dimethyltryptamine and other tryptamine derivatives, are able to cross the blood-brain barrier through active transport, thus making them valuable targets for LNP derivation. These NTlipidoids have been combined with brainimpermeable LNPs to produce blood-brain barrierpenetrating LNPs.
In this manner, a drug carrier previously unable to access brain tissue is chemically modified to enable this access Once inside brain tissue, lipid degradation and biomolecule release occurs This method has been successfully utilized to release amphotericin B (an antifungal) into the brain, and is currently being further developed for chemotherapeutic applications [12]
In conclusion, glioblastoma is a devastating illness that is extremely difficult to treat for a variety of reasons, the primary of which is low accessibility of deep brain tissue, which experiences rapid and pervasive invasion by tumor cells, resulting in an extremely poor prognosis. Nanotechnological advances, particularly the development and adaptation of Lipid Nanoparticles (LNPs), are a highly promising solution given their biochemical versatility, biocompatibility, and biodegradability. LNPs have a high capacity of precision, enabling them to target tumor cells while minimizing the cytotoxic effects characteristic of traditional chemotherapies. As a result, there are diverse avenues, all with major potential to revolutionize cancer treatment, being explored to harness LNPs using a range of techniques, structures, and targets, to effectively address the genetic and molecular heterogeneity of glioblastoma tumors in a manner that is safe, accessible and effective.
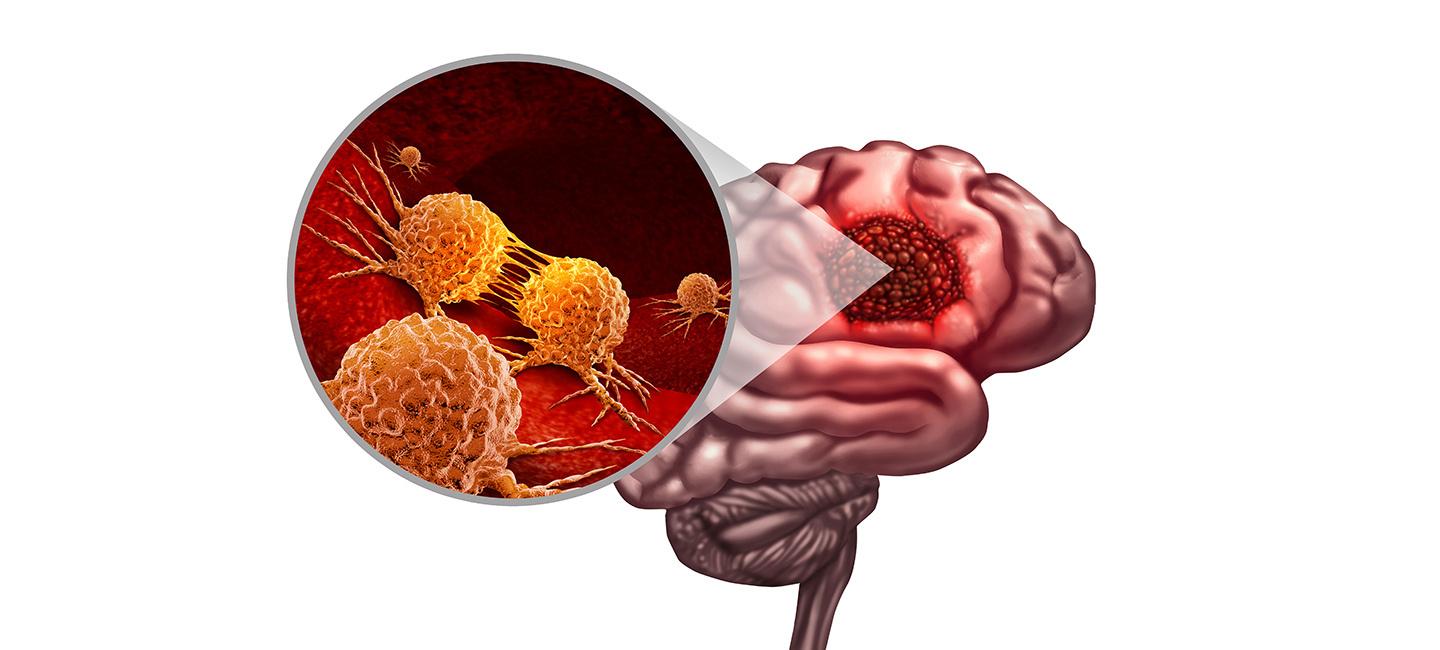
The Challenges with
CRISPR-Cas9 Technology
Written by Anna Shell Edited by Saraswati Sridhar Designed by Jenny LiCRISPR-Cas9, the novel genome editing technology, has revolutionized medicine However, with great power comes great responsibility,anddiscussionssurrounding the technology contain healthy doses of optimismandskepticismalike Issuesof
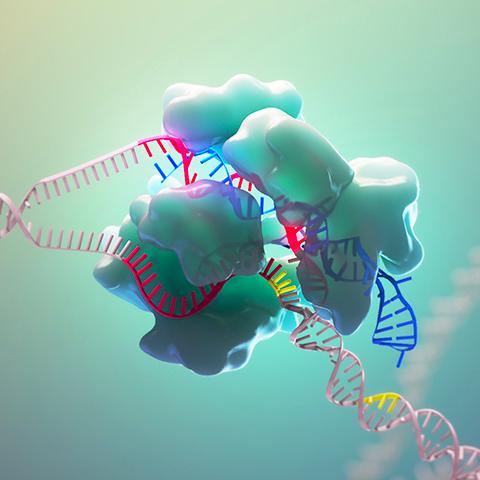
ethics and access are at the forefront of these conversations, both within the medical community andbeyond
In 2018, just three years after the successful demonstration of CRISPR with eukaryotic cells [1], a Chinese doctor by the name of Dr He Jiankui announced his utilization of CRISPR to confer HIVresistance to twin girls [2] Waves of backlash ensued, with Dr Jiankui ultimately facing 3 years in prison and a fine of $430,000 [3] Some, such as bioethicist Jonathan Kimmelman, hoped the scandal would prompt global conversations; he wascorrect[4].
Publicconcernimmediatelyturnedtoso-called “designer babies,” embryos edited for aesthetic or intellectual purposes. Beyond this consensus, however, opinions are divided. Only 53% of those polled in the United Kingdom in March 2022 would be in favor of genomic editing to help eliminate severe, life-threatening conditions; only 36% would be in favor of intervention for manageable conditions.
In a November 2021 Pew study, only 30% of Americans thought the widespread use of gene editing to reduce a baby’s risk of developing serioushealthconditionswouldbeagoodideafor society While 30% thought it would be bad for society, 39% were unsure Furthermore, 55% of Americansthoughtsucheditingwouldincreasethe gap between higher and lower income Americans, with35%thinkingitwouldn’tmakemuchdifference and8%thinkingthegapwoulddecrease[5].
Dr. Peranteau, Attending General, Thoracic, and Fetal Surgeon at Children’s Hospital of Pennsylvania, expressed concerns over designer babies. Nothing is free, he explains, both from a monetary and risk perspective, and weighing risks versus benefits is a vital part of medical interventions. Thus, he says, it is totally unethical to accepttherisksassociatedwiththesetherapiesfor aesthetic benefits. He also explains how many physical traits, such as eye color, are polygenic, and thus more complex. Even with monogenic traits,thereisriskwiththemultiplicityofonegene’s functions Dr Jiankui’s controversial study involved asinglebasepairdeletiontoconferHIV-resistance However, this isolated modification also increased risk of infection from West Nile virus, influenza A, andtick-borneencephalitis[6] Weighing the risks and benefits of gene modification, even beyond aesthetic purposes, is anticipated to be a massive hurdlewithCRISPRtherapies
Anotherethicalconcernsurroundsheritability Dr Peranteau outlines how fetal gene editing, as it stands, focuses on mid-to-late gestation fetuses, different from embryonic editing performed on a single-cell embryo The goal, he explains, is not to change the germline and pass modifications to future generations. Dr. Anne Muigai, who served on a WHO Expert Advisory Committee assessing the need for governmental oversight of genome editing, touts the primacy of somatic gene editing therapies, involving non-reproductive cells [7]. Any edits to these cells would not be passed on, avoidingheritabilitychallenges[8].
A potential avenue for reducing the costs of CRISPR-based therapies involves allogeneic treatments, or “off-the-shelf” treatments
Allogeneic therapies involve taking cells from a healthy donor, editing them to confer the desired genetic change, then cloning them into large batches for multiple recipients [11]. Ongoing trials, such as those at CRISPR Therapeutics [12], show incredible promise; the potential for such treatments in the field of cancer immunology is staggering. Currently, Dr. Stadtmauer explains, immunotherapies take 1-3 months. Allogeneic therapies could be administered in a week. The most important considerations, he explains, are that the T-cells and the cells of the host don’t recognize each other as foreign, and that the Tcellsareabletocarryoutpotentattacks.Although there is no allogeneic therapy currently FDAapproved, current trials are incredibly encouraging
It is up to governments to ensure ample oversightofanynewtechnologytomitigateissues of both ethics and access However, neither of these challenges can dampen the raw excitement surroundingtheimplicationsofCRISPR-Cas9
Alongside ethical concerns, there is the issue of access CRISPR therapies are incredibly expensive and few and far between The price tag on current genetic therapies for blood disorders range between $1-$2 million CAR T-cell treatments, involving engineering immune cells to attack cancerous cells [9], are incredibly effective; yet, a 2021 study estimated that costs for such therapies canrangefrom$700ktoover$1million[10].For12% of the patients in this study, costs exceeded $1 million.“Theworldisinsane,”saysPennMedicineDr. Stadtmauer, “and I just try to make it sane one patient at a time.” Dr. Stadtmauer uses CRISPR to improve the potency and efficiency of CAR-T cell cancer immunotherapy. Sometimes we conflate charges with costs, he says, and this is not a physician’sissue,butapolicymaker’sissue.Itisthe job of legislatures and private firms to ensure the insurance industry is caught up to covering new therapies Dr Stadtmauerwon’tinitiateatreatment unless he is confident that it will not pose a financial burden to the patient Penn, he explains, currently has a program assessing the financial toxicity of some of these therapies Step one is to identify which patients face the most financial hurdles, an essential duty of a comprehensive cancercenter
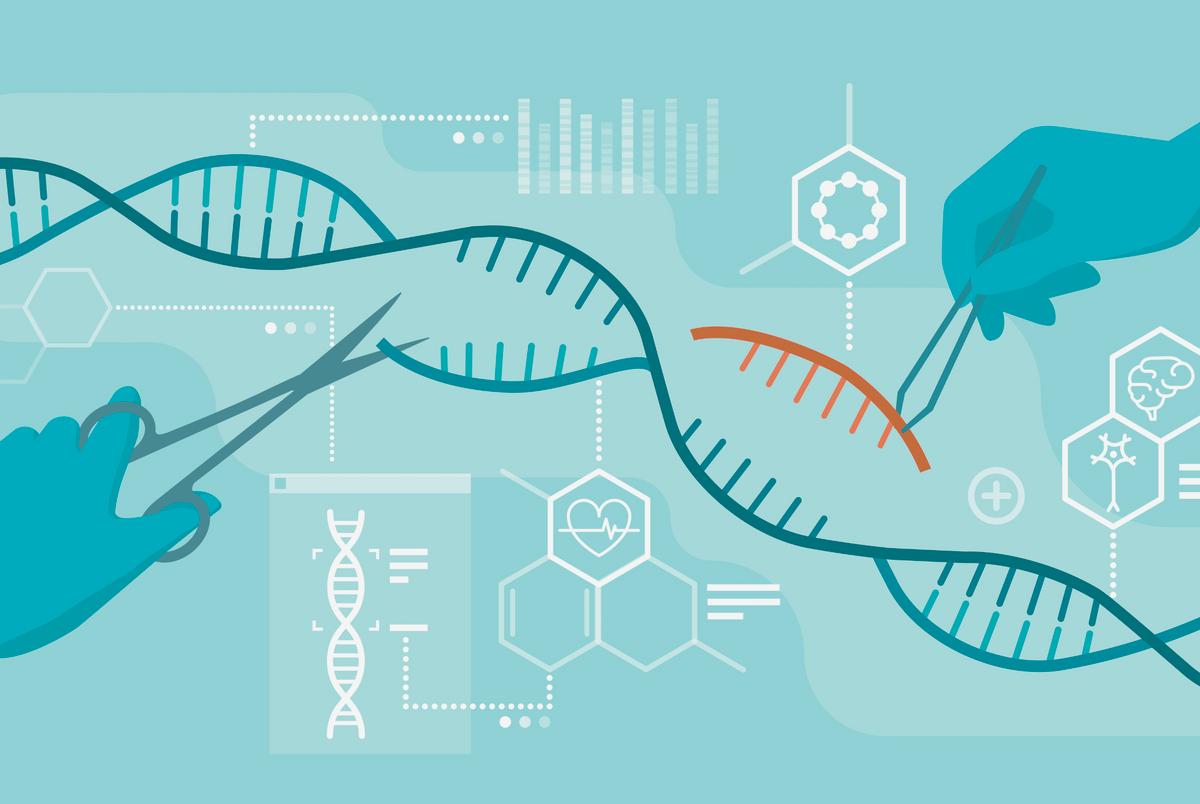
"Nothing is free, both from a monetary and risk perspective, and weighing risks versus benefits is a vital part of medical interventions."
CARS DRIVE INNOVATION IN DEADLY DISEASE.
Written by: Eric Lee Edited by: Aarsha Shah Designed by: Siri DanduSeven-year-old Emily Whitehead had little hope left. She suffered from fatal relapsed acute lymphocytic leukemia (ALL), a cancer that caused her to bruise and bleed excessively, and almost underwent hospice procedures due to her condition’s severity. However, in 2012, things turned around—Whitehead was miraculously saved. Enter the heroes of this story: a team of ambitious scientists and their mighty CAR-T cells.
CAR-T cell therapy is the product of decades-long progress in cancer therapy research. In 1989, Israeli immunologists Zelig Eshhar and Gideon Gross engineered a T cell with a chimeric antigen receptor (CAR)—a laboratory-made protein designed to bind to cancer cell membrane proteins called antigens. When CARs bind to cancer cell antigens, an activating signal is sent to the T cell, triggering a molecular cascade that causes the death of the cancer cell. Eshhar and Gross’s initial discovery was then followed by several subsequent iterations of CAR-T cells using gene-editing techniques, with each iteration having greater antitumor potency than the last. CAR-T cells made major headlines in the scientific press as a result of a 2012 clinical trial, the very trial in which Whitehead’s life was saved. In this study, T cells were harvested from blood through leukapheresis, modified with CARs that target the leukemia antigen CD19, and reinjected into the body [1]. As a result, Whitehead’s immune system developed a stronger response, which was sufficient to reverse ALL’s progression and cure the disease.This clinical breakthrough was made at the University of Pennsylvania by a team of immunologists led by Carl June, Bruce Levine, David Porter, and Stephan Grupp. Because of the overwhelming positive outcome of the CAR-T clinical trial, the field of CAR-T therapy has experienced massive growth and has reached and benefitted even more patients [2]. Currently, the FDA has approved five CAR-T cell therapies, including Kymriah, the regimen tested by June’s team in 2012. All of these therapies target the CD19 or BCMA antigens and treat lymphomas, multiple myeloma, and autoimmune conditions like lupus [3].
Because CARs can theoretically be engineered to bind to any antigen, cancer is not the only illness whose treatment could benefit from CAR-T cell research. When combined with mRNA technology, CAR-T cells can repair tissues in the body. For example, at Penn’s Perelman School of Medicine, researchers modified T cells to target and eliminate fibroblasts, which cause heart tissue to scar and hasten the onset of heart failure. This research used the same antigen-targeting strategy of cancer CART cell therapy: a gene encoding a T-cell receptor that binds to antigens on cardiac fibroblasts (fibroblast proteins) was inserted into the T-cells, and subsequently used to target the fibroblasts. Just as CAR-T cells that fight blood cancers hone in on tumors, these anti-fibrotic CAR-T cells are able to identify and kill fibroblasts. However, what distinguishes anti-fibrotic CAR-T cells is the method by which they are produced: instead of being harvested from the patient and transformed outside of the body, these cells can be modified in vivo. Moreover, the mRNAs are short-lived, which causes CAR-T cells to revert back normal T cells after a few days of sufficient fibroblast depletion and allowing fibroblasts, which are crucial in tissue regeneration, to survive [4]. Despite CAR-T cell therapy’s wide-ranging possibilities, the field still has many obstacles to surmount, one of which is its lack of universal efficacy. The most notorious side effect of CAR-T cell therapy is cytokine release syndrome (CRS), the onset of inflammation caused by the rapid release of cytokines from activated immune cells. Because the severity of CRS is directly
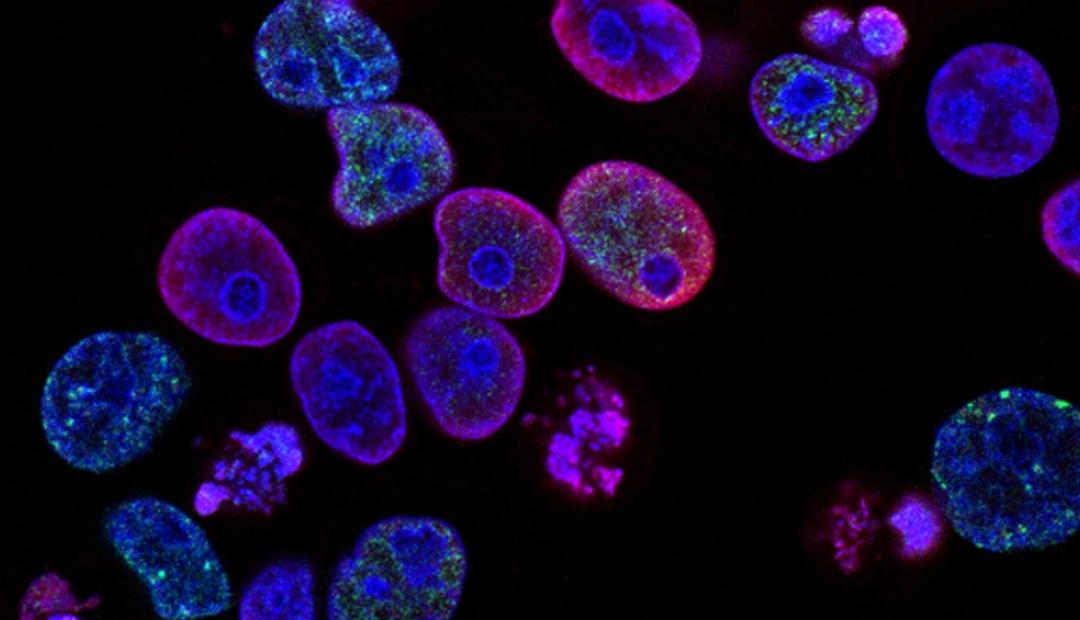
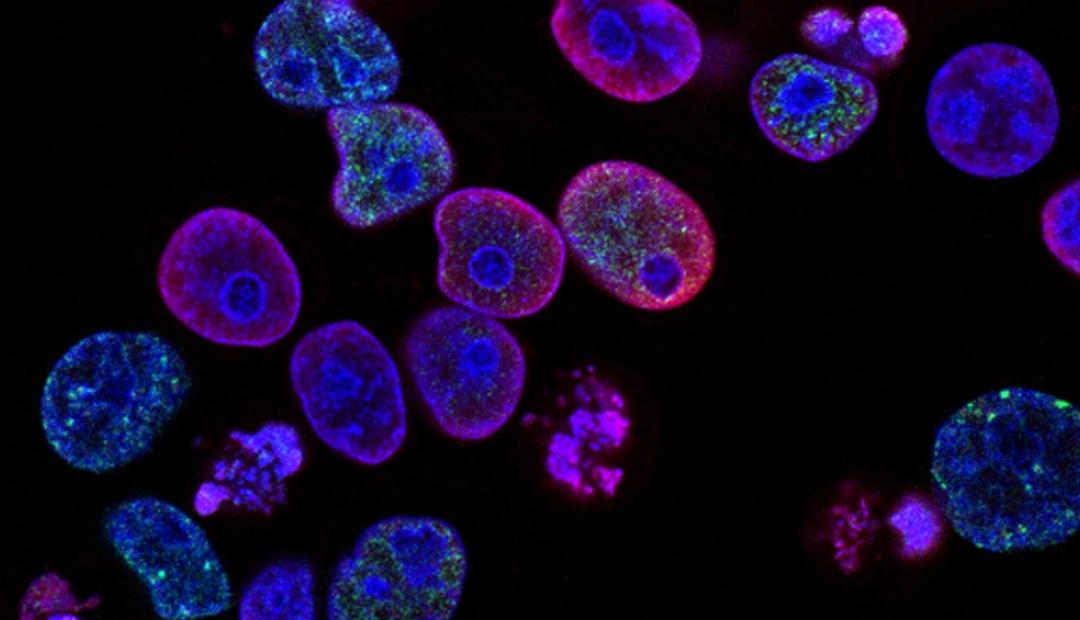
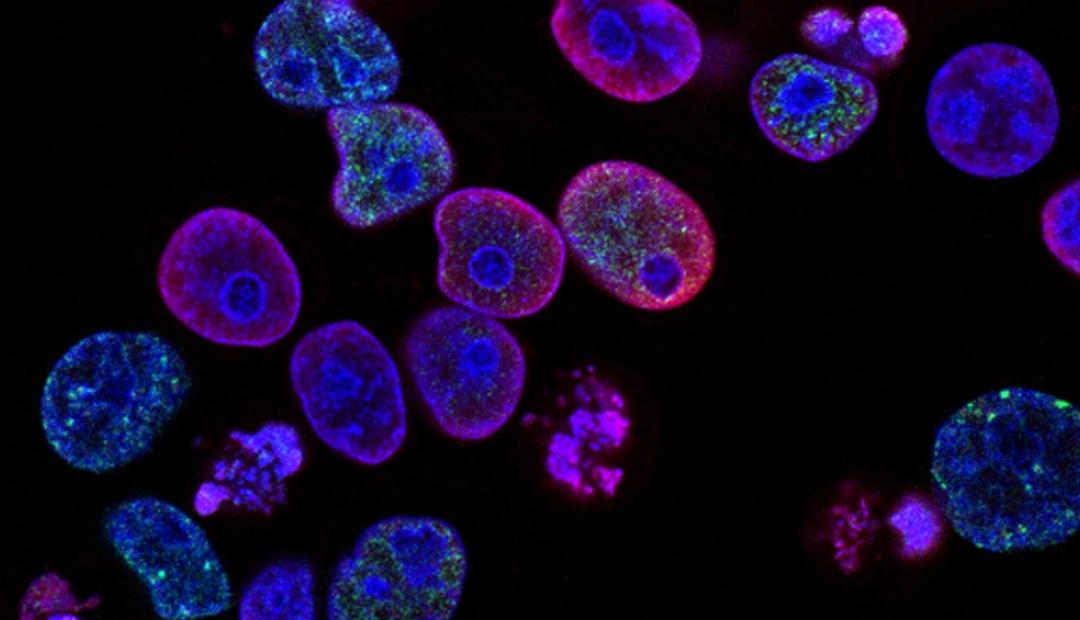
Figure 1: CAR-T cell therapy is when T-cells are genetically modified to express MHC-independent antibody binding domains on their surface, allowing them to recognize and kill cancer cells. Courtesy of Tej Patel.
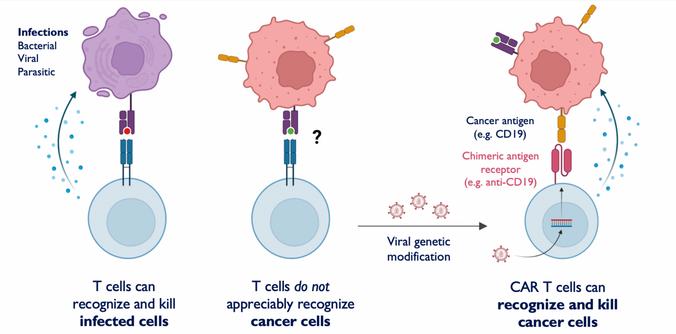
correlated with the activity of T cells in the body, patients with the most advanced forms of cancer experience the most acute CRS symptoms [5]. Although monoclonal antibodies and steroids may be used to mitigate CRS symptoms, these treatments may reduce the efficacy of CAR-T cell therapy. If CRS is instead allowed to progress unhindered, patients bear a higher risk of mortality, as CAR-T cell therapy has an associated lethal toxicity over ten times greater than that of other immunotherapies [6].
CAR-T cell therapy is challenging to employ in the treatment of solid tumors such as lung and breast cancers, although it is effective in treating blood cancers. This can be attributed to the difficulty of identifying antigens on such tumors that are different from those of healthy cells, as a result of a phenomenon called tumor heterogeneity, in which solid tumors display vastly different antigens and are difficult for T cells to access. A lack of chemokine expression and presence of dense tissue surrounding solid tumors render conventional CAR-T cells useless. Another factor that deters the widespread use of CAR-T cells in cancer treatment is the tumor microenvironment, in which immune cells like regulatory T cells produce growth factors and checkpoint inhibitors that enhance tumor growth and propagation. When combined with reactive oxygen species (ROS), checkpoint inhibitors block the activity of other immune cells like T cells. Thus, although conventional CAR-T cells have a higher activity level than normal T cells, they are still subject to influences from the tumor microenvironment [7].
Another major barrier present in CAR-T cell therapy is cost. For example, due to the complex logistics of harvesting and modifying patients’ T cells, Kymriah carries a price tag of half a million dollars. Operations and management procedures following treatment cost up to another half million [8]. ICANS and CRS were the primary driver of longer hospital stays for CAR-T cell therapy patients. To manage these conditions, patients must receive seizure prophylaxis and immunosuppressive drugs, which further contribute to the cost of care. This issue is further exacerbated by nonmedical costs, such as those associated with long traveling distances and treatment times, which may incur significant travel costs and loss of earnings. From the provider’s standpoint, thanks to the cost of having trained specialists provide treatment and maintain the necessary capacity to keep patients in hospital beds, hospitals are allowed to charge up to five times the price of the drug, which inflicts an even greater cost burden upon the patient. Although CAR-T cell therapy is more expensive than other existing treatments, it has been proven to result in better health outcomes [9]. This results in a difficult decision for patients, who are forced to make tradeoffs between treatment quality and effectiveness and cost-reduction.
Although CAR T-cell therapy is associated with many challenges, new advances in the field offer promising solutions. Some researchers are avoiding problems associated with CRS by targeting cancers located in the brain, where immune cells are not active. By targeting a protein associated with gliomas, CAR-T cells can fight brain tumors without provoking a flood of cytokines from the rest
of the immune system [10]. In other research, the National Cancer Institute has created a different type of CAR that can reduce CRS and ICANS symptoms in CAR-T cell therapy patients by making CAR-T cells secrete less cytokines, reducing toxicity without compromising the quality of treatment [11]. Newly researched molecular “switches'' composed of genes that trigger cell death when expressed can also allow clinicians to stop overactive CAR-T cells and reduce cytokine concentrations if CRS and ICANS symptoms become too severe. In contrast, another type of CAR-T cell uses cytokines to overcome the immunosuppressive conditions of the tumor microenvironment, allowing the cell a way to fight solid tumors.
New CAR-T cell research can make the creation and administration of treatment less expensive for hospitals and patients. There are two strategies that scientists can use to avoid harvesting T cells from patients and culturing them ex vivo, which carries high labor costs and is not always effective. The first is the utilization of lipid nanoparticles to deliver gene-editing tools to T cells inside the patient’s body, as has been done to treat cardiac injury by editing T cells to target fibroblasts. The problem that remains for researchers to solve is enhancing the longevity of T cells edited this way, as the gene-editing tools delivered by lipid nanoparticles are short-lived. The second strategy is to treat patients with off-the-shelf CAR-T cells, which patients can receive anywhere without undergoing time-consuming apheresis. Because allogeneic CAR-T cells are foreign and face the risk of an immune response [12], off-the-shelf CAR-T cells can be derived from the patient’s own induced pluripotent stem cells (iPSCs). These iPSCs are cultured in conditions similar to those of the thymus, where T cells grow, and have been shown to expand much more rapidly and produce more robust T cells than conventional T cell cultures [13]. Furthermore, for treatment to be affordable to patients, policy solutions must be combined with scientific innovation. U.S. Medicare and Medicaid have agreed with Novartis on a deal based on an outcomesbased model, in which services rendered for administering Kymriah are only paid for if the patient responds within thirty days [14]. In addition, bundled payments systems, in which paying healthcare providers for treating the patient’s disease holistically instead of paying for individual services, may cut costs by incentivizing providers to focus on outcomes, streamline operations, and minimize unnecessary services [15].
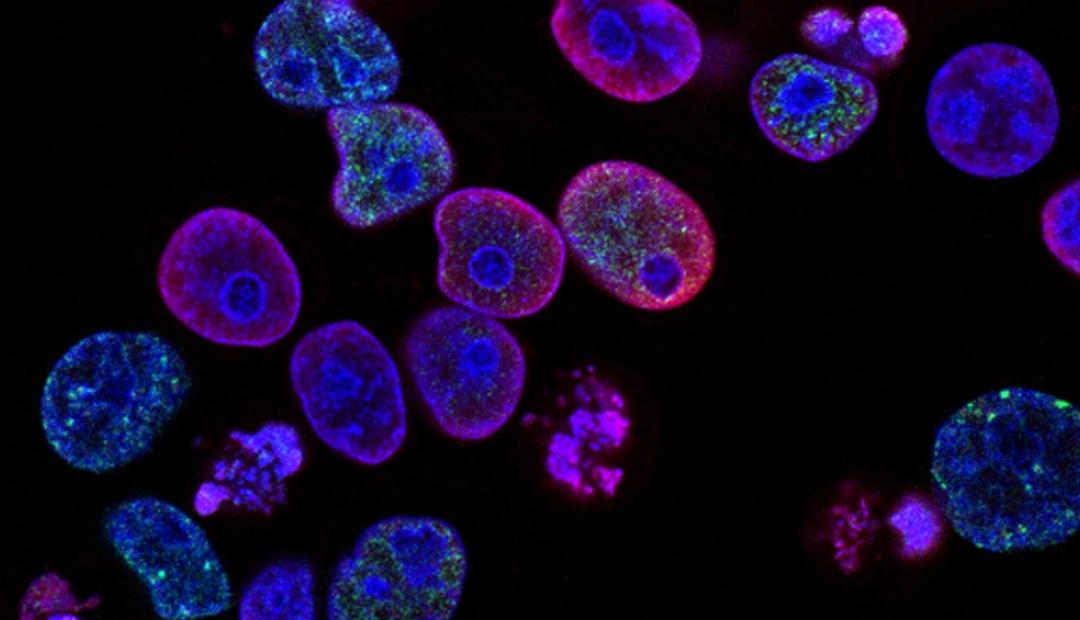
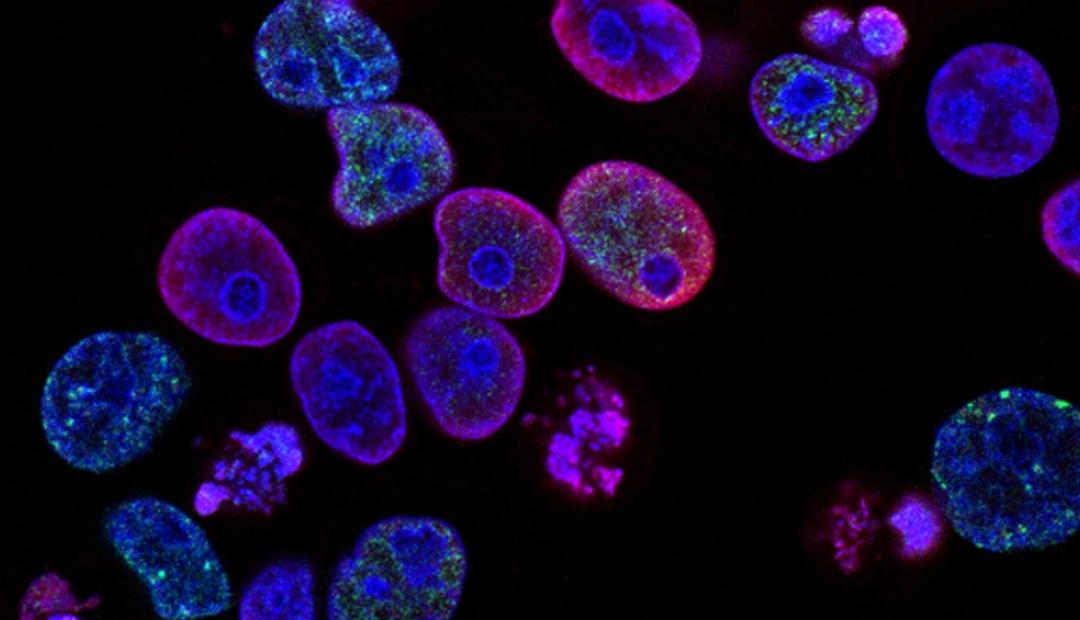
A future driven by CAR-T cells is a future in which there is new hope for patients with advanced cancer, autoimmune disorders, tissue diseases, and more. Although CAR research is relatively new, it has already yielded many lifesaving therapies and holds promise of novel, accessible, and effective treatment for a wide variety of illnesses. Consequently, CAR-T cell therapy may become the next gold standard of treatment in oncology and beyond if it becomes affordable to the average patient, which may become possible with such a large amount of talent, funding and enthusiasm, bringing new hope for a next generation of medical treatments for devastating illnesses.
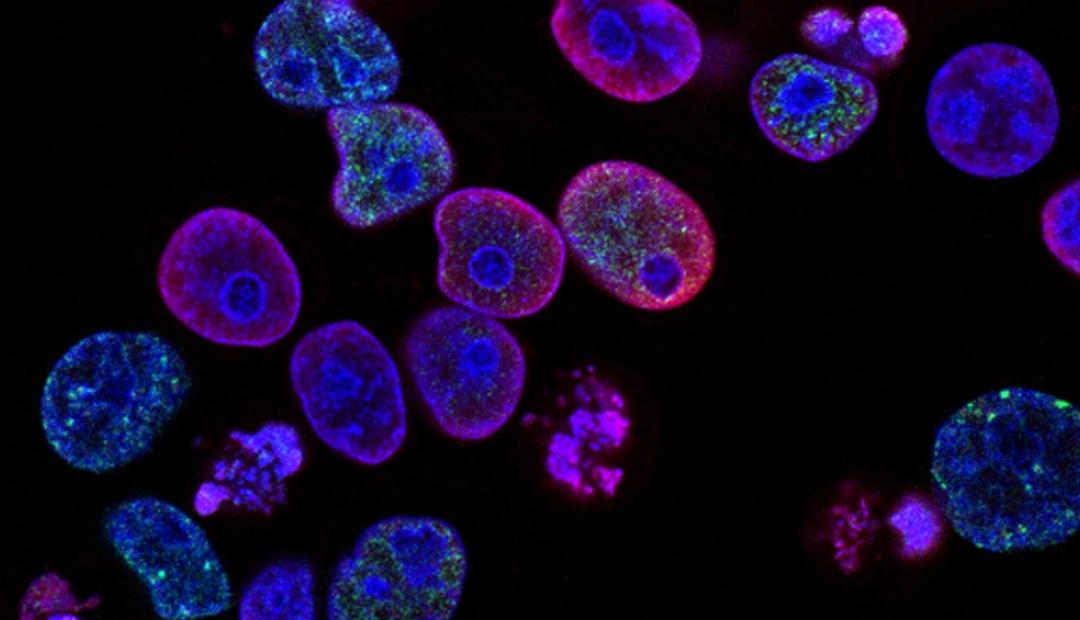
Revolutionizing Vaccines: The Development and Significance of mRNA Technology
Written by Jaskeerat Gujral Edited By Jerry Cai Designed by Laura JannettaIntroduction
Everyhighschoolbiologyclasstouchesuponafewcore topics:ecology,genetics,cellularrespiration,amongothers.Yet notopicismorewidelyrecognizedasthecentraldogma—theway ourcellscreateRNAfromDNAandthentranslatethatRNAinto protein.MessengerRibonucleicAcid,alsoknownasmRNA,isa crucialpartofthatdogma.mRNAisessentiallyashort-livedcopy ofaportionofDNAthatencodesforaparticularprotein.Atfirst glance,itseemssimpleenough,butthissimplestringofcodehas beenakeyplayerinvaccinetechnologyusedtofightagainst COVID-19andhashelpedmillionsacrosstheworld[1].
HistoryofmRNAandEarlyDevelopment
ThemRNAmoleculewasfirstdiscoveredinthe1960s.Soon afterthissignificantdiscovery,researchersusedliposomes, whicharefattymembranestructures,totransportmRNAinto murineandhumancellstoinduceproteinexpression.The primarygoalofthistechniquewastohavetheliposomesserveas amediumforgeneticmaterialtotransferintothecell.Since then,therehavebeenmanystudiesthathaveaimedtoleverage mRNAfortherapeuticuses.Challengesconstantlyarosesince mRNAwasunstableandexpensivetoworkwith.Thereweremany academiclabsthatexperienceddifficultyindeterminingthe accurate“formula”forthenucleicacidsandfatsthatwouldaidin developmentofthetechnology.
Twodecadesago,Dr.KatalinKarikoandDr.DrewWeissman decidedtocollaborateanddevelopthemRNAtechnology.Dr. KarikoiscurrentlythecurrentseniorvicepresidentofBioNTech, aGermanbiotechnologycompanythatfocusesondeveloping immunotherapies.Dr.DrewWeissmanisthedirectorofvaccine researchandtheInstituteforRNAInnovation.Initially,Dr.Kariko was involved in research in the neurosurgery department, researchinghowshecouldutilizeRNAtotreatneurological conditions,suchasstroke.Dr.WeissmanwasatPennestablishing his own immunology lab. Around 1997, Dr. Kariko and Dr. Weissman decided to collaborate to create a vaccine for HIV/AIDS.Dr.KarikoworkedwiththemRNAaspect,whileDr. Weissmanwouldworkfortheimmunologicalsideofthevaccine. Inoneofherstudies,Dr.Karikoobservedhowinflammatory responseswereinducedinmicewhentreatedwiththesynthetic
mRNAthatsheutilizedinherpriorstudiesFollowing this,thepairobservedthatthemiceexperienced harmfulinflammatoryresponses,causingthemiceto die[2] BothDr KarikoandDr Weissmanrealized thatthecausefortheexhibitedreactionwasthat conceptthatthemRNAwasreacting withTolllikereceptors,whicharereceptorsthatfunctionas firstresponderstodangersignalsfrompathogens. Toll-like receptors are crucial in regards to inflammation because they mediate immune responsestowardsvariouspathogen-drivenligands [3].Dr.KarikoandDr.Weissmanwereconsistentand dedicatedtodetermininghowtheycouldprevent thisinflammatoryresponse.
Fastforwardto2005:Dr.WeissmanandDr. Karikodeterminedthattherearrangementofbonds on uridine to create pseudouridine caused the immunesystemtoinhibitanauto-immuneresponse. Overtheyears,manyresearchersunderestimated thepotentialofthisscientificdiscovery,butitledto BioNTechandModernashowingsignificantinterest inthepair’sresearchdiscoveriesSubsequently,the majorcompanieslicensedtheirpatentsandutilized theirresearchtodeveloptheCOVID-19vaccines. Thetwocompaniesledthefurtherdevelopmentand production of the vaccines that have been distributedallaroundtheworld.
ApplicationsofmRNATechnology
mRNAtechnologycanbeusedtotreatawide spectrumofconditions,suchascancer,neurological conditions, cardiological conditions, and genetic diseases[4].
Specifically,mRNAtechnologyiscurrentlybeing used to develop vaccines for many infectious diseases, including HIV, hepatitis, malaria, and tuberculosis;thesearesomeoftheleadingcausesof deathsthroughouttheworld.Developingvaccines wouldhelpthosewhosufferfromthesedeadly conditions.
Intherealmofcancerresearchandtherapy, manyresearchersandacademiclabsaredetermined tofindasignificanttreatmentorcure.However,the functionofvaccineswouldbealittledifferent. Generally,vaccinesareusedtoprotectindividuals fromacertainvirus.Whendealingwithcancer, however, the mRNA vaccine would serve as a treatment option to induce a strong immune responseforonetohavetheirowncellstoattackthe cancerouscells[5].Throughextensiveresearch,it was discoveredthatthemRNAvaccinehasthe abilitytoinduceastrongantibodyresponsetofight againstcancercells[6].Themajorgoalofthisformof
vaccine would be to develop into a form of personalized medicine, similar to Chimeric antigen receptor T-cell (CAR-T) therapy, a form of treatment that researchers utilize to cause T-cells to induce a cellular response to target cancerous cells. The mechanism of action is to obtain a patient's T-cells through a blood sample and genetically modify them in a laboratory setting to allow them to attack cancer cells According to Katharina Reinhard, the director of Immunoreceptor Therapy at BioNTech, there are promising results that show the efficacy of RNA vaccines in conjunction with CAR-T cell therapy to elicit a stronger immune response Currently, there are several cancer mRNA vaccine clinical trials that are being monitored [7] There are several studies that show the potential of mRNA vaccines treating cancer because of their ability to elicit an immune response without causing toxic effects [8]
mRNA vaccines are also being developed for genetic disorders, specifically for cystic fibrosis (CF). CF is a progressive genetic disorder that causes an individual to produce large amounts of viscous mucus and accumulate in the lungs and pancreas; CF induces inhibition of cystic fibrosis transmembrane conductance regulators (CFTR). The downstream effect would allow chloride ions to rush into the cell, preventing cells from staying hydrated and causing thick mucus to be produced. Researchers have two major ideas as to how they can utilize mRNA vaccines to effectively treat CF: one is to deliver mRNA into the lungs, where lung cells can initiate production of healthy CFTR, and the second is to alter the DNA sequence that ultimately fixes the CFTR protein [9] Through additional research, mRNA vaccines could provide a breakthrough treatment option for several genetic disorders, such as CF
Overall, mRNA technology has been proven to be crucial in the scientific and medical world It is the reason as to why the world was able to get through the pandemic that left the world in isolation and longing for a solution Researchers are further determining its applications to other conditions; hopefully, this promising technology can treat other serious conditions.
The Biomechanics of Synthesized Tissue: A Study of Stem Cells in Tissue Engineering
Written by ARJAN KAHLON Edited by MEGAN ZHANG Designed by JANINE NAVALTAAs of early 2023, there were over 100,000 people on the organ transplant waiting lists in the United States alone, with a new individual added every 10 minutes. Despite each donor’s ability to save up to eight lives and “enhance” over 75 more, an average of nearly twenty Americans die every day waiting for an organ transplant [1] The ratio of patients on the waiting list to transplants performed for vital organs is skewed far from one to one, and this imbalance is growing worse with time. Recent advances in tissue engineering now may allow us to ask: instead of transplanting entire organs from donor to recipient, what if we could provide safe and effective treatment without requiring an organ donor at all?
Tissue engineering involves the process of generating live tissue that can carry out the functions of its natural counterpart, allowing it to replace damaged or diseased tissue within living organs Clinically, tissue engineering provides hope for possibly correcting a wide range of diseases while improving function and quality of life for patients. As the practice and implementation of tissue engineering are refined and synthesized organs are perfected, this novel approach could significantly reduce the growing need for full-organ human transplants, thus addressing several currently massive burdens on the U.S. healthcare system: costly organ rejection treatments, expansive treatment of opportunistic infections in transplant patients, and backlogged, often in-equitable, transplant waiting lists.
HOW DOES TISSUE ENGINEERING WORK?
The generation of synthetic tissue starts with the basic functional unit of life: cells The first step in tissue engineering is the identification and isolation of the stem cells needed to form a target tissue. In their natural growth and development process, stem cells differentiate into various types of specialized cells, given the correct environment and signaling molecules, making them the ideal basis for ‘growing’ a preselected type of new tissue. Samples of stem cells can be obtained from different types of sources, including allogenic sources (a donor of the same species) or heterologous sources (a donor from a different species altogether, often bovine) The preferred stem cell sampling method, however, is from an autologous source–a specimen from the patient themselves. If possible given situational factors such as the patient’s baseline health and risk of infection, the autologous method circumvents the body’s natural immune systems and greatly reduces the occurrence of donor tissue rejection. Such rejections of non-autologous transplanted donor cells can have catastrophic impacts on a patient’s body; thus the current standard of care with allogenic transplants requires costly, preemptive, and sometimes perilous immunosuppressive ...
therapies [2] These therapies suppress the recipient's natural immune system to reduce the risk of rejection of allogenic donor tissue. Immunosuppression unintentionally increases the patient’s susceptibility to “opportunistic infections,”[3] which are triggered by commonly present microorganisms that are typically neutralized by a healthy patient’s immune system These risks are heightened during and immediately after the treatment process, given the patient’s increased exposure to transmissible diseases commonly present in healthcare facilities. This weakening of the immune system is also associated with increasing patients’ susceptibility to a range of non-infectious diseases, including certain cancerous and metabolic diseases.
The next step, after harvesting and isolating the appropriate stem cells, is to seed them onto a scaffold, which is a synthetic form that mimics the architecture of the target tissue and serves as the physical backbone for the development of the engineered tissue. The scaffold, made of biocompatible materials such as collagen or synthetic polymers, provides a rigid physical structure calibrated to enable stem cells to attach, grow, specialize, and eventually proliferate [4] Because stem cells differentiate into a variety of specialized cells to form diverse tissues throughout the body, certain signals will guide them in specific directions of differentiation in the scaffold and stem cell matrix By mimicking natural structures, for example, the scaffold shape may provide mechanical signals to ensure proper stem cell differentiation [5]. In addition, some types of scaffolds are composed of materials designed to degrade slowly over time, allowing the newly differentiated cells to fill in the gaps left behind and eventually support the structure of the engineered tissue by themselves. In this way, the desired architectural structure of the newly grown tissue is preserved as the biocomposite scaffold deteriorates [6] and is replaced with new, live tissue.
In addition to mechanical signals, specific biochemical ................
markers and signaling molecules are utilized to ensure that differentiation produces the desired cell type, and thus forms functional tissue [7] Growth factors and nutrients are also essential for the rapid growth and development of large numbers of differentiated cells from an initial sample of comparatively few stem cells [8], [9]. Thus, a variety of nutrient and protein-related molecules in the form of growth factors and hormones are added to the culture, prompting appropriate cellular differentiation and later, the growth and proliferation of those cells. The presence of the proper quantity and type of these molecules is paramount to the efficient development of functional tissue, and therefore, varies widely depending on tissue types and the unique needs of each patient.
heart disease, and osteoarthritis Chronic disease involves degeneration and loss of the function of vital tissues, and accounts for nearly 75 percent of aggregate U.S. healthcare spending, according to the CDC [11]. As modern medicine has developed treatments for the symptoms of many of these diseases, the most advanced treatments currently involve the use of pharmaceuticals, corrective surgery, radiation, or transplant surgery, all of which are not always effective and often have side effects. Tissue engineering would provide patient-specific cures–beyond just the treatment of symptoms–for many types of chronic disease, including many which are not amenable to organ transplants.
Currently, human organ transplants are one of the few options which offer complete and thorough solutions to the underlying cause of certain diseases; however, the scarcity of donor organs means this solution is costly and limited. With long waiting lists, the transplant lists are imperfect, inequitable, and untimely, with many patients waiting years to move to the top of a transplant list for certain high-demand organs Unfortunately, many patients become increasingly ill or die before receiving their much-needed transplants. The supply and demand aspect of organ transplantation, which has developed into a widely bemoaned flaw, places massive emotional strain on patients and their families, and poses a significant financial and logistical burden for healthcare providers and institutions alike.
These three essential components–stem cells, scaffolds, and signaling molecules–are brought together in a bioreactor, a controlled setting that allows the targeted culturing, growth, and development of tissue [10]. Environmental conditions such as temperature, humidity, acidity, and even oxygen levels are tightly controlled within the bioreactor to facilitate cell development and proliferation along the scaffold as desired Each of these variables changes between use cases as the most favorable conditions are customized based on the needs of each specific tissue. This closely guided cell growth and differentiation fill in the scaffold, yielding functional tissue that eventually can be harvested for transplantation into a patient to replace damaged or diseased tissue.
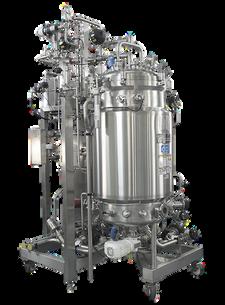
IMPACT ON HEALTHCARE SYSTEM
The integration and implementation of tissue engineering techniques have the potential to revolutionize many aspects of the modern healthcare system The impacts of these changes range from patient-directed solutions that result in quicker and more successful outcomes for the individual patient, to more broad impacts on the US transplant system, including avoidance of costly organ rejection treatments, treatment of chronic infections in transplant patients, and the lightening of the transplant waiting lists.
The use of successfully refined tissue engineering techniques in replacing diseased end-stage tissue extends an exciting promise towards curing chronic diseases such as diabetes, .........
With the potential to impact patients at a rate and volume unhindered by the supply of donor organs, tissue engineering offers the opportunity for a massive improvement in both the quality and speed of treatment of many diseases The possibility of regenerating or replacing ailing tissue with advanced tissue engineering techniques provides a unique treatment option that might not only eliminate the underlying drivers of the disease but also could prevent the resurgence of chronic diseases in treated patients If the most critical tissues within organs, or even entire organs themselves, could be replaced with synthesized tissues, the massive burdens of chronic disease and transplant on the healthcare system would be alleviated. Tissue engineering would allow for a simultaneous reduction of the demand for organ donors, an increase in patient treatment rates, and, most importantly, a meaningful increase in the quality of life for patients.
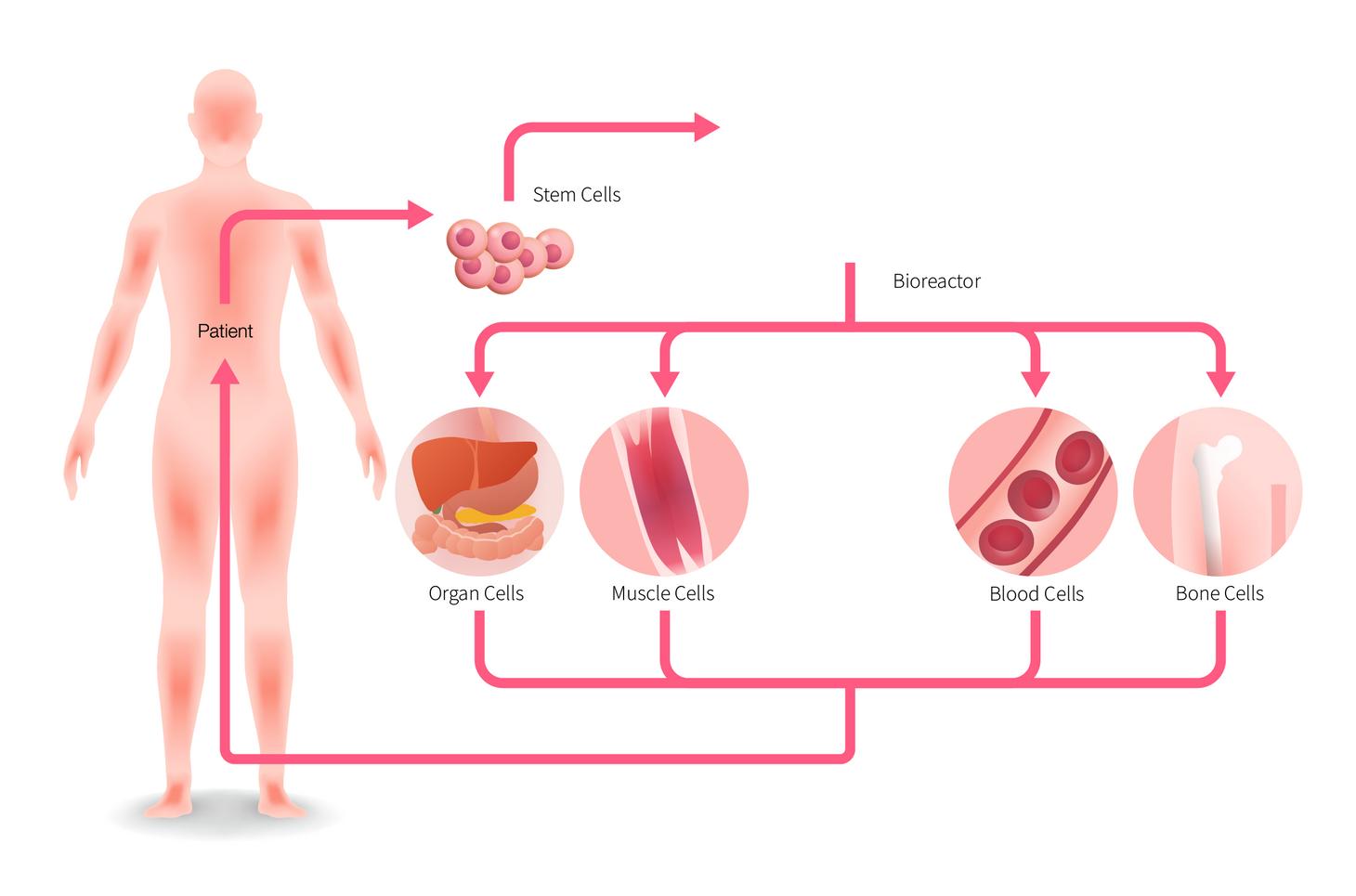
THEORGANCRISIS ANDHOWARTIFICIAL ORGANSPRESENTA SOLUTION
Afteracuteorganfailure,modernmedicinehasfewviablelongtermtreatmentoptionsoutsideofseekingoutanorgandonorfor a transplant. With not enough donors to match the growing demand,patientswhourgentlyneedanorgantransplantareleft with no choice but to join a waitlist. This organ shortage is life threatening:7,000to8,000peopledieeachyearintheUS alone becausetheyaren’tabletoreceiveadonororganintime[1] Many patientsdelayregisteringorareunawarethattheyneedtoregister, causing the breadth of the organ donor pool to be less than it otherwisewouldbe[2].
Theeffectsofthiswidelyfeltcrisisdisproportionatelyimpactthe poor Despitepotentiallyexperiencingalowerseverityofillness, wealthierindividualshaveahigherlikelihoodofreceivingadonor organbecausetheycanaffordtoenterthewaitlistmultipletimes, whichcanbethedifferencebetweenlifeanddeath[3].
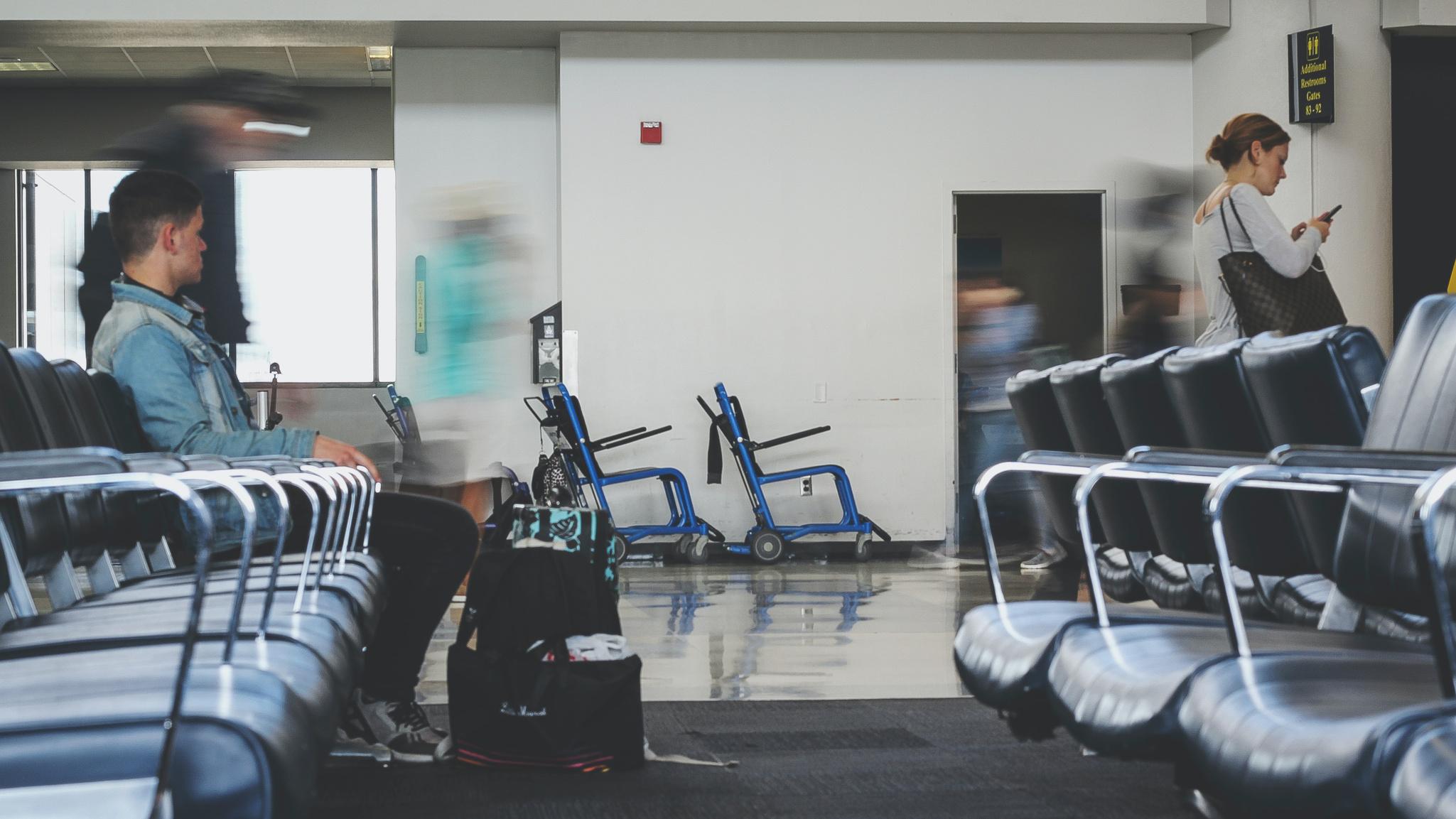
As the crisis continues to worsen, laws have been proposed to providepotentialremedies Somemeasuresincludealteringorgan donation policies to make registering simpler and less timeconsuming, increasing educational awareness through government-funded programs, and developing additional organ procurementinfrastructure[4] Thesemeasuresmayhavemerit:a study by the World Health Organization concluded that historically,countriessuchasSingaporeandChilehavebeenable to increase the supply of organs by passing donor prioritization policiestoincentivizedonation[5].
Written by NOLAN ANTHONY Edited by ARJAN KAHLON Designed by JANINE NAVALTAThe most controversial solution, presumed consent laws, “ presumes”(withtheoptiontoopt-out)thatanymemberofthe generalpublicautomaticallygivesconsentfororgandonationin the event that they cannot make the decision themselves. In contrast,theU.S.,alongwithmanycountriesincludingtheU.K., Germany,andSwitzerland,useopt-inorgandonationpolicies,in which the general public instead must consciously opt-in to the organ donation system [6] The impacts of this policy might initiallyseempositive:ameta-analysisof58studiesconductedby Dr. Jon Jachimowicz, a professor at Harvard Business School, confirmed that people tend to stick with the default option, indicatingahigherorgansupplywithapresumedconsentpolicy [7].Crucially,however,WesterncountriesliketheUnitedStates tend to prefer individualism, which adds nuance to the implementationoforganpolicies.Infact,theNationalAcademy of Medicine concluded in a study that people in the US are actuallymorelikelytobecomedonorswhentheyhavecontrolof thatchoice,ratherthanthegovernmentmandatingthattheydoso [8] Empiricsdemonstratetherisksofpresumedconsent:Nevada State Representative David Orentlicher wrote that although the U.S. attempted presumed consent on a limited basis for forty years; the policy ultimately failed because family members were afraid that the presumed organ donor would not have wanted their organs removed [9]. Thus, successful case studies of presumed consent from other countries are not necessarily indicativeoftheireffectivenessintheUS
Ethical concerns about presumed consent center around the supposedriskofcatastrophicallyinjuredpatientsbeingviewedless aspatientsintheirownrighttobetreatedtothefullestextent,and more as “ organ farms,” referring to the potential of their viable organs to treat other patients [10]. Specifically, 92% of organ donors are brain-dead individuals, despite only being a small portionofthosedying.Critically,thecriteriausedtodetermine death for “heart-beating organ donation” are flawed, and could potentially be fatal for a “patient whose condition is otherwise salvageable”Thisisaseriousdilemmaandcanleadtounnecessary donor death: 60% of heart-beating donors who matched the clinicalbasisofbraindeathwerelaterdetermineduponautopsy that their condition was recoverable [11] If countries were to imposepresumedconsentlaws,thekeyquestionthenliesinwhat criteria they would use to ensure that hospitalized patients are treated to the fullest extent possible before being considered as candidatesfororgandonation.
FIG. 1: Image of a waiting room [27]Fortunately, a potential solution to the organ shortage is in the midst of being developed: man-made artificial organs The first successful artificial organ transplant took place in 1982, during which researcher Robert Jarvik and inventor Willem Kolff implantedanartificialheartinahuman[12].Kolffalsocreated the first functioning dialysis machine and heart-lung machine, leadingtohisreputationas“thefatherofartificialorgans.”
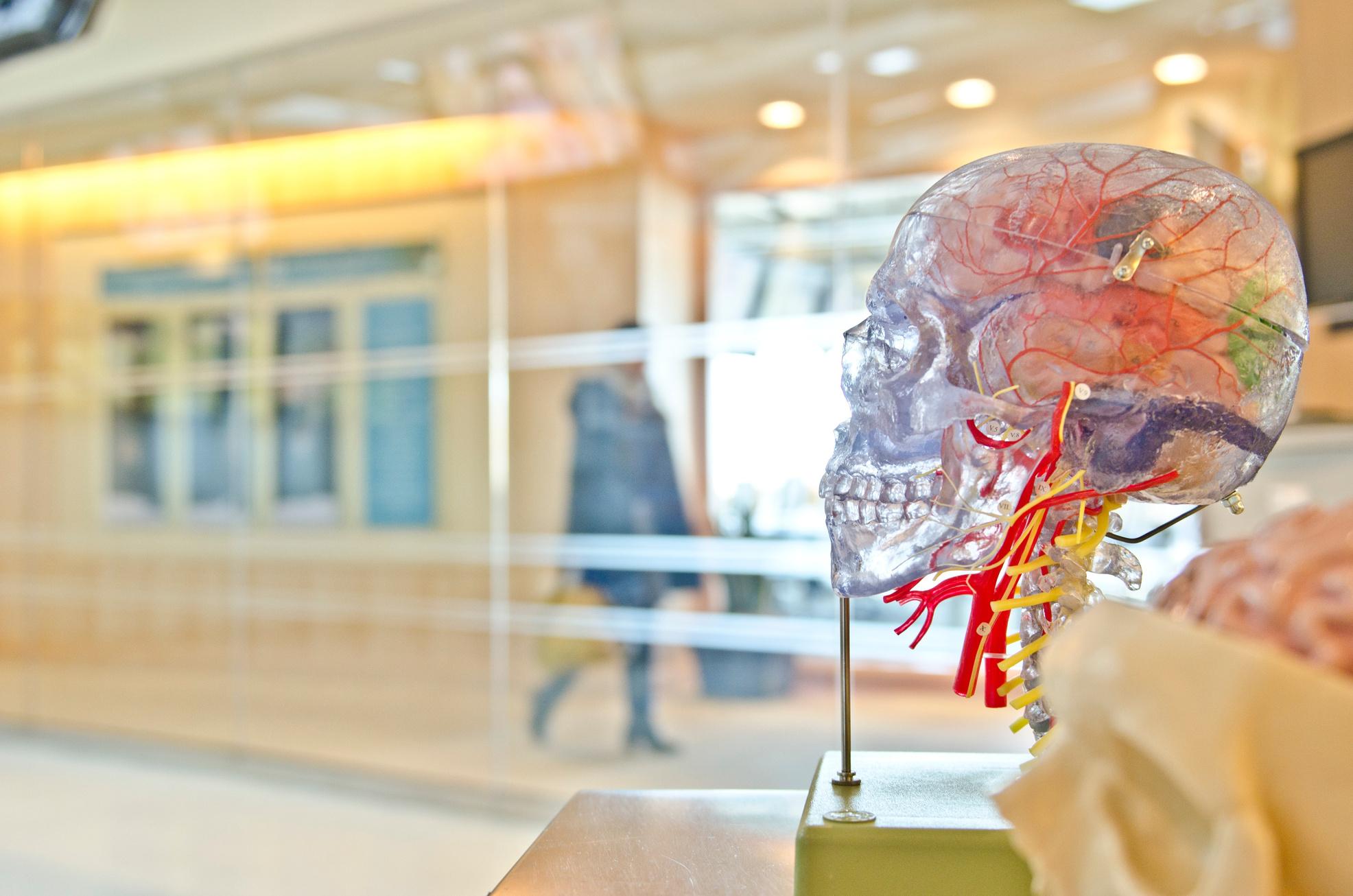
Thefirstmethodtoproduceman-madeorgansisthroughtissue engineering, where scientists regenerate organs through a combination of growth factors, living cells, and synthetic materials The second is the utilization of stem cells, where researchersgrowareplicaoftheoriginalorgan Inthepast,these reconstructions have been mostly limited to skin, but advanceshaveallowedscientiststoexpandintootherreg thebodylikethethymus[13].Third,throughorganbiop the process of using 3D printing technologies that m different cells and biomaterials to create a close replica original organ [14] Artificial organs have also been de throughmachinelearningandartificialintelligence[15] networksidentifyandproducecellstructureswhichcan used for research on complex diseases like macular degen [16] The artificial organ market, currently worth rough billion, is expected to continue to grow at a 7.5% com annual growth rate to reach over $28 billion in 202 Nevertheless, cost remains a significant barrier: a 3-D organ transplantation, for example, can cost hundr thousandsofdollarsforasinglepatient[18].Pricesfora organswillneedtofallbeforetheycanbefeasiblyimpleme alargerscaleandincreaseaccess Yetdespitethehighcosts,experts such as renowned surgeon Stephen Westaby have affirmed that therapidpaceofinnovationinartificialorganscouldpotentially easedemandfortransplantswithinseveraldecades[19]
Beyond promising treatment possibilities for those currently on transplant waitlists, addressing the organ shortage has value in other
other areas as well One such benefit is reducing kidney dialysis usage. Kidney dialysis is the process of removing excess solutes, fluids, and toxins from the blood to prevent buildup in failing organs. In the U.S., many citizens receive dialysis through Medicarebecausedialysisisessentialbothinsupportingpatients withkidneydisease(theninthmostcommonsourceofdeathin the U.S.) and keeping patients on the organ transplant waitlist alive,withimpactsrangingfarbeyondindividualhealthoutcomes Forexample,asinglepatientondialysiscostsanaverageestimate of$14millionover5-10years,[20]whichhasimplicationsonthe national scale; by some estimates, costs associated with dialysis treatment alone accounted for 20% of Medicare’s funding in a single year [21]. If dialysis funding was able to be scaled back, Medicare could provide higher quality care for its 44 million recipients, cover more patients, or reduce healthcare spending altogether.
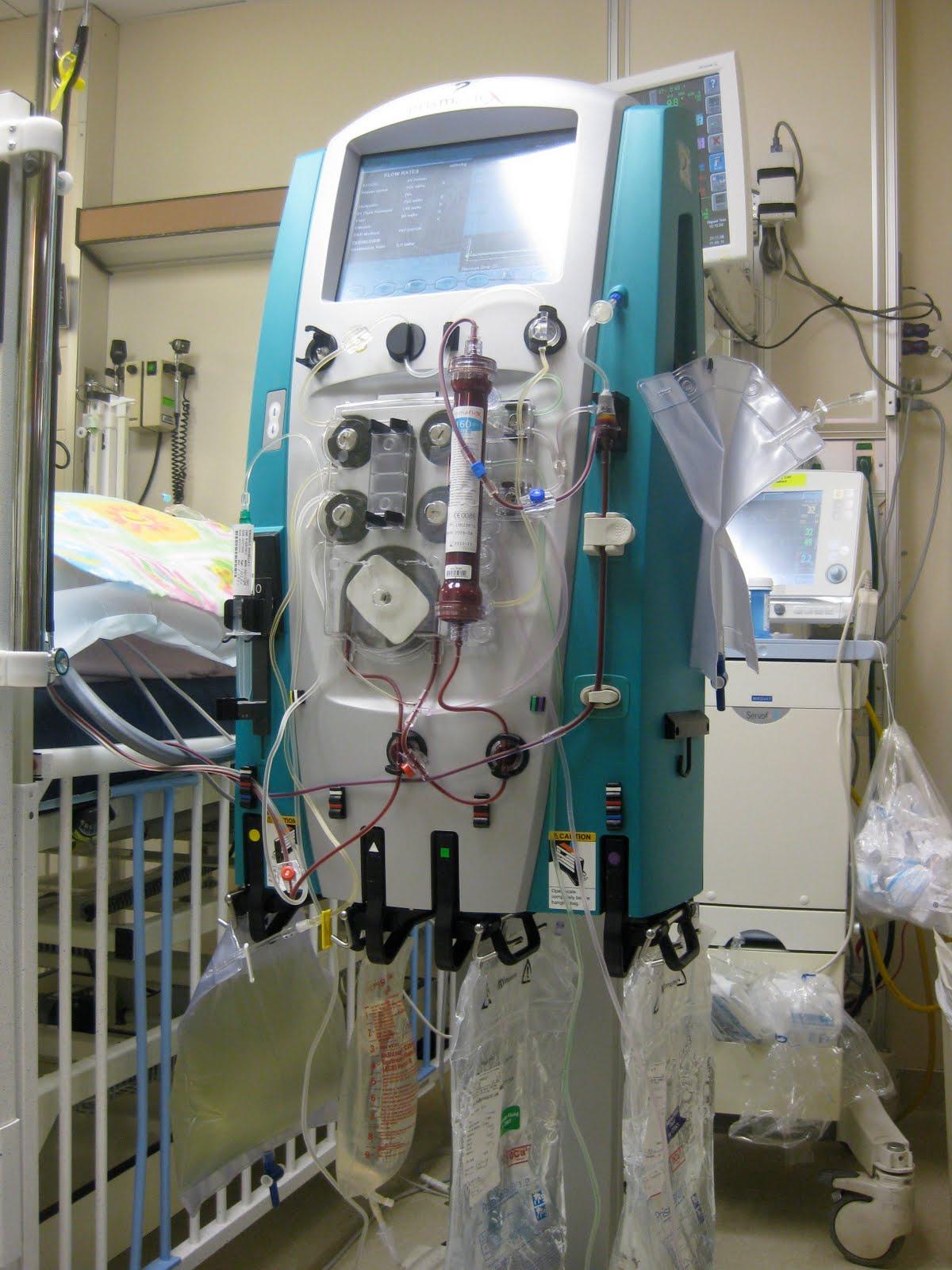
Anotherbenefitisaddressingorgantrafficking Organtrafficking is a lesser discussed form of human trafficking because of its intricateandoftenstealthynature,despitestillaccountingfor10 percent of all organ transplants [22] The organ shortage, and resultingincreaseinorgan-failurerelateddeaths,hasprompteda recentriseinorgantrafficking,[23]with11,000organstakenin oneyearalone[24].Organtraffickinghasballoonedtothepoint where the United Nations recognizes it as a cause of human traffickingasawhole[25].Toeffectivelydetergrowthoftheblack market,TheDukeGlobalHealthInstituteconfirmsthatcountries mustfirstincreasethelegalorgansupply[26]
Theorgancrisis’sconsequencesonlivesandcostshasproliferated legal and ethical concerns throughout the world, yet not much progresshasbeenmade Whileartificialorgansremainasolution indevelopment,theirrapidpaceofinnovationandmarketgrowth offersanopportunitytoendthecrisisandpreventsimilarimpacts inthefuture.
FIG. 2: Image of a dialysis machine [28]Exciting Ways of Reducing Excitation: Stem Cell Therapy and its Antiepileptic Potential
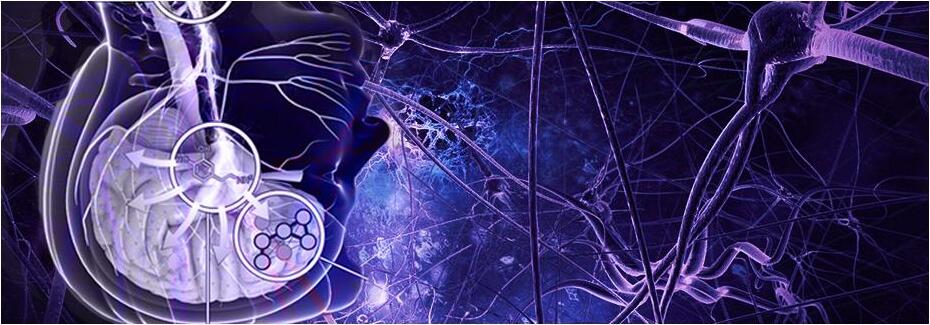
Emerging advances in stem cell research show promise for treating a wide range of disorders and injuries such as Alzheimer’s and Parkinson’s diseases, spinal cord injury, and epilepsy. The aim of this article is to highlight the potential of regenerative medicine for epilepsy, a chronic neurological condition characterized by recurrent seizures that are triggered by excessive electrical discharges in neurons, abnormal patterns of mossy fiber sprouting, and hippocampal sclerosis (loss of neurons) [1]. While the causes of these seizures are often unknown, several factors including genetics, infections, immune system dysfunction, or previous injuries (strokes, trauma, tumors, etc.) give rise to its etiology. Among the 50 million patients globally, approximately two thirds suffer from focal, rather than generalized epilepsies, which are most commonly located in temporal lobes (TLE) [2].
The most well-supported explanations for the pathogenesis of epilepsy are recurrent excitation or recurrent inhibition. Hyperexcitable dentate granule cells of the hippocampus have been found to play a pivotal role in the onset of seizures. In epilepsy patients, the mossy fibers (axons of the dentate cells) form abnormal connections with other neurons and thereby form malfunctional, hyperexcitable circuits. Moreover, the loss of inhibitory neurons in the hippocampus further promotes seizure initiation and propagation. There is plenty of evidence from human patients and animal models in support of both mechanisms.
The TLE model resembles many patients’ pathological conditions, including cell loss of excitatory neurons in discrete hippocampal regions, decrease of inhibitory gammaaminobutyric acid positive (GABAergic) interneurons, formation of abnormal neuronal circuits, and changes in expression level of several receptors and ion channels. dFinally,
hippocampus is disrupted with subsequent hyperexcitability of the hippocampus [3].
Indeed, numerous studies suggest that increasing GABA, the most prevalent inhibitory neurotransmitter, lowers the risk of seizures [4]. Additionally, emerging literature suggests the existence of a bidirectional relationship between inflammation and the development of seizures. An increase in proinflammatory mediators, which are created by the immune system in response to injury or other damage, eventually leads to leukocyte migration and conformational changes of endothelial cells, which cover the blood vessel inner surface. The alteration of the tight junctions ultimately results in bloodbrain barrier leakage and the recruiting of proinflammatory cytokines. It has also been proposed that extracellular potassium levels rise in these conditions, which increases hyperexcitability and susceptibility to seizures [5].
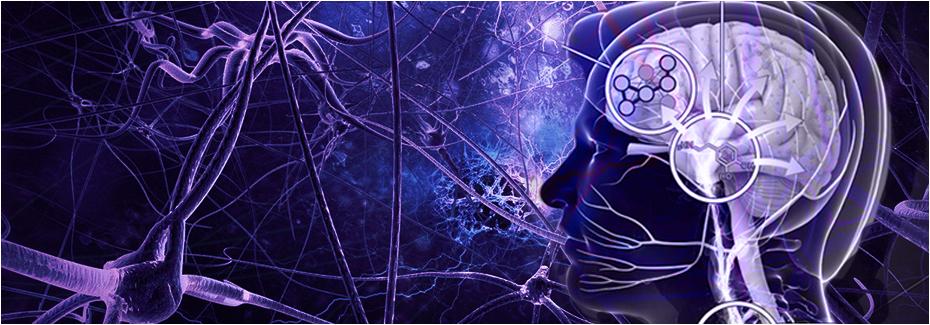
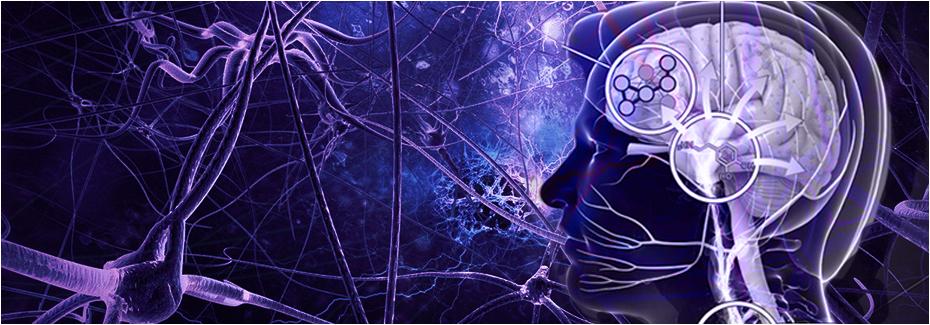
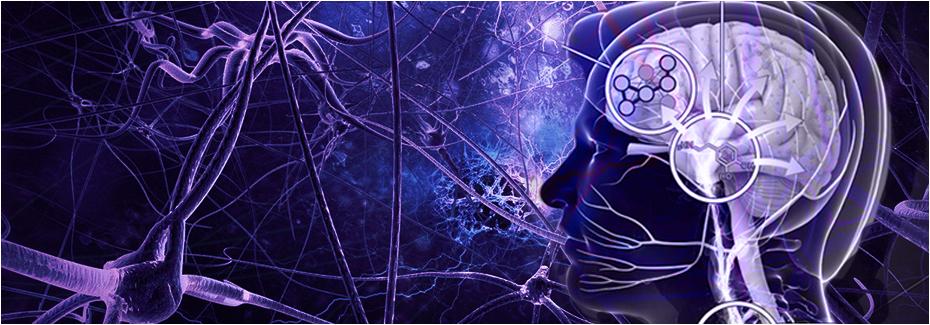
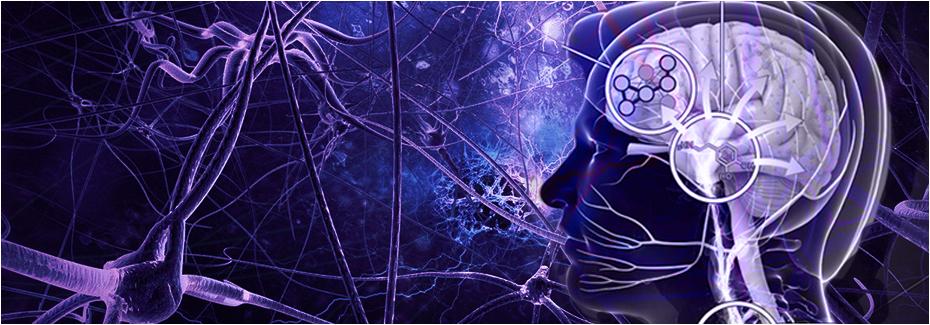
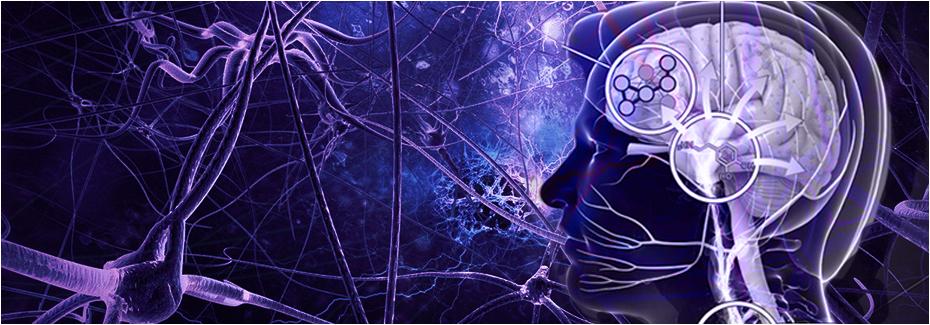
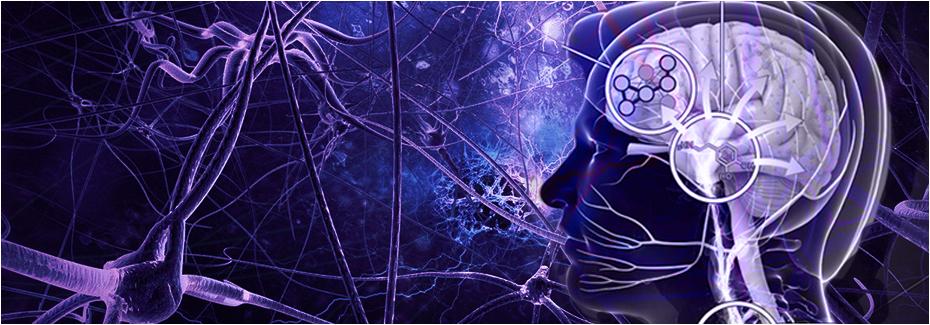
Currently, most medications work by inhibiting sodium channels or activating GABA receptors to reduce neuronal excitability. Less common treatment methods like deep brain stimulation or surgical removal of affected areas are deployed to ameliorate some of the symptoms. Unfortunately, around 30% of patients remain refractory, or resistant, to antiepileptic drugs, highlighting the need for more efficacious interventions [6].
A growing body of research suggests that regenerative medicine may redefine the future of epilepsy prevention and treatment. Stem cells are adult cells with self-renewing capacities, which can help repair damaged tissue. Notably, stem cell therapy utilizing mesenchymal (MSC) and embryonic stem cells (ESCs) has already been shown to improve treatment outcomes for several neurodegenerative diseases. MSCs can be easily derived
adipose tissue [7]. They can differentiate into different lineages of mesenchymal tissue, including chondrocytes, osteoblasts, and adipocytes, or into further cell types such as neurons, cardiomyocytes, and endothelial cells. ESCs operate in a similar manner. In the context of epilepsy, different subtypes of transplanted stem cells exert their therapeutic effects through mechanisms like the replacement of lost GABA neurons, the reshaping of the inhibitory neural circuitry as well as substitution of physiological mediators in immunomodulatory and anti-inflammatory processes.
For instance, in-vitro studies have shown that MSCs can modulate the implicated inflammatory response via the release of anti-inflammatory factors. Peripheral organ damage upregulates proinflammatory cytokines in blood, ultimately reaching the brain. Their subsequent interaction with a neurovascular unit (structural and functional interactions between brain cells and blood vessels) reduces the neuronal excitability threshold. MSCs inhibit the pro-inflammatory leukocytes at the site of damage and release various factors that maintain endothelial integrity from cytokine interactions. Furthermore, MSCs can switch macrophages from type 1, which is proinflammatory, to type 2, thereby promoting an anti-inflammatory phenotype. NSC transplantation can also create new astrocytes, which are crucial for the expression of the glial-derived neurotrophic factor with anticonvulsant properties [8].
Similar properties make ESCs a viable candidate for epilepsy treatment. Studies done on the hippocampi of mice have shown that ESC transplantation in vitro results in successful differentiation into neural progenitor cells and enables the replacement of various inhibitory interneurons [9]. Remarkably, the effects of NSC transplantation appear to be long-lasting, with a survival rate of 30% three months post-grafting. Out of those, over 90% successfully differentiate into inhibitory mediators. These GABAergic interneurons have also been shown to express markers such as neuropeptide Y, which is implicated in the inhibition of glutamatergic excitation in the dentate gyrus of the hippocampus [10].
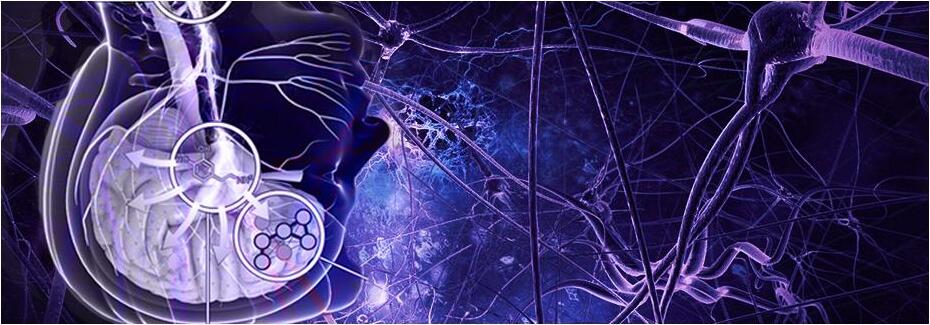
Another promising mechanism of action is focal adenosine augmentation, which suppresses seizures either via adenosine kinase inhibitors or adenosine receptor agonists [11]. Alongside its neuroprotective properties, adenosine is known to act as an anticonvulsant. In rodent studies, a genetic disruption of the adenosine kinase, the adenosine metabolizing enzyme, has been shown to increase adenosine release and aid its antiepileptic role [12].
Given that stem cells can be obtained from both autogenic and allogenic sources with relative ease and administered via noninvasive routes, we are hopeful that this field will reveal important findings with direct clinical applications in the near future. While the majority of research on regenerative medicine pertains to animal models, a number of preclinical human trials have shown promising results. Consequently, various dfdafdafdsafdsa
researchers are now opting for model-oriented approaches to gain deeper insight into the perturbations of the pathological neuronal networks that play a key role in epileptogenesis. In the upcoming years, we may observe the creation of both 2D and 3D organoids derived from induced pluripotent stem cells (iPSCs). These produce complex oscillatory waves and may be used to explore the nature of glutamatergic and GABAergic signaling in epilepsy [13].
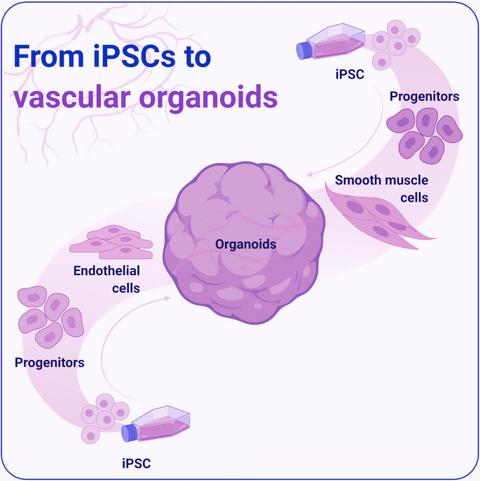
The current trajectory of epileptic care is shifting from symptom mitigation to addressing the roots of epileptogenesis, bringing hope to currently treatment-resistant patients. This improved understanding, coupled with new discoveries within molecular biology and s increased quality and availability of brain imaging and EEG analysis methods, has enabled scientists to create more accurate prediction models and treatment methods. Despite its obvious benefits, the potential risks associated with stem cell therapy are a definite concern. For example, retroviral application in iPSCs could lead to uncontrolled differentiation and viral contamination, which may give rise to tumorigenesis. Therefore, more longitudinal research on the long-term effects of stem cell transplantation should be conducted in order to develop safe and efficacious therapies [14]. Should these efforts prove to be fruitful, stem cells may soon usher in a new era of antiepileptic treatment and transform the lives of millions of people around the world.
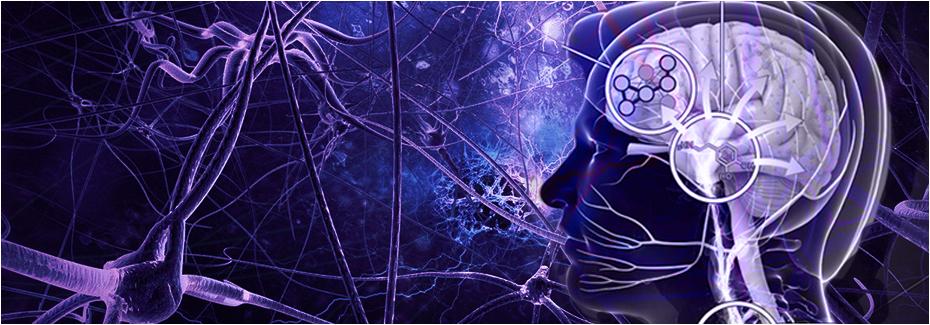
StemCell Therapeutics for Traumatic BrainInjuries
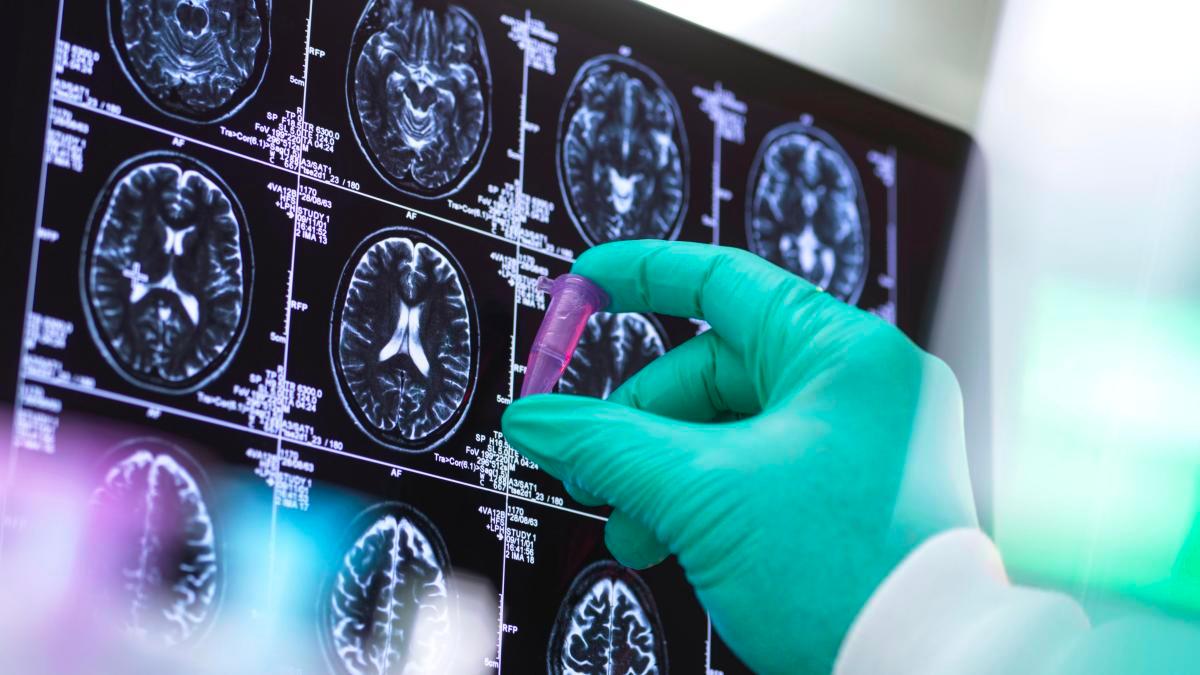
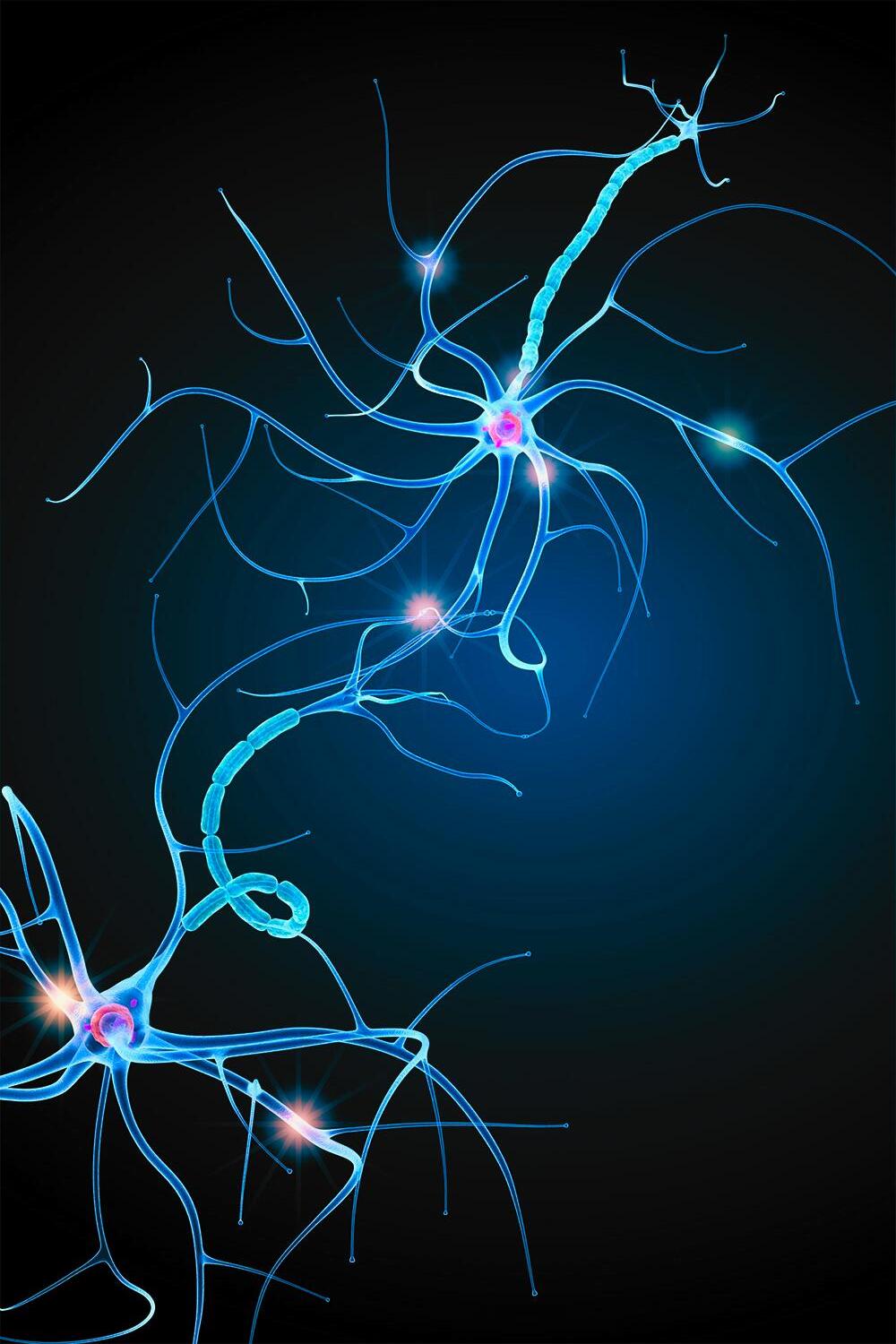
Introduction
Traumatic Brain Injuries (TBIs) are prevalent in everyday life with approximately 28 million people suffering from a TBI each year [1] However, many athletes participating in high-contact sports are unaware of the detrimental and potentially life threatening consequences that TBIs have After NFL fans witnessed Miami Dolphins’s Tua Tagovailoa suffer multiple concussions and lose consciousness, mild TBIs (mTBIs) were in the spotlight for the rest of the season Changes to NFL protocols were made accordingly, yet many still lack knowledge on the severity of symptoms and the therapeutic interventions for treatment of mTBIs
What are TBIs?
A person suffers a TBI when they endure a jolt towards the head or neck, which can inflict varying brain tissue damage depending on the severity of the biomechanical force TBIs can initiate widespread neuroinflammation, axonal dysfunction, neurodegeneration, and deterioration of the blood-brain barrier [2] Unlike other physical injuries, neurotrauma has multifactorial implications unseen by the naked eye, leading to a myriad of possibilities for symptoms and ambiguity in the timeline for recovery and diagnosis On the other hand, concussions present in different ways, leading to potential delays in treatment Moreover, the delay can be increased depending on whether the athlete can accurately self-report their symptoms in a timely manner The repercussions for patients who prolong the treatment process by self-reporting later are grave If patients incur another TBI, the severity of symptoms may intensify, triggering life-altering chronic conditions An aggregation of TBIs could even lead to chronic traumatic encephalopathy, a progressive neurodegenerative disease that inflicts memory loss, aggressive and unstable behavior, and severe cognitive impairment [3]
With such serious consequences associated with TBIs, therapeutics must be both effective and time-efficient while also remaining dfsfddf
affordable. Depending on the severity of the TBI, various therapeutic interventions may be recommended. For physical manifestations such as skull damage, blood clotting, internal cerebral bleeding, surgical methods can be used for repair. Equally important, intangible issues and internal dysfunction must also be addressed. If a patient experiences psychological distress or emotional imbalances, therapy serves as an excellent alternative. Speech, cognitive, and physical therapy may also aid recovery of basic functions.
Stem Cells
Despite the aforementioned therapeutic interventions, there is no true effective drug treatment for TBIs [4] However, recent advances within stem cell therapy that aim to mitigate induced, irreversible neuronal loss seem promising Known therapeutic stem cell treatments were extended for TBIs and even conducted across various animal models The afflicted area can experience different forms of neuronal deficits in the cerebral cortex which can ultimately disrupt neural networks and render them dysfunctional Depending on the injury site, the types of neuronal
loss in the cerebral cortex can be cell-specific Some types of affected neurons include glutamatergic, dopaminergic, GABAergic, or even NG2 cells, and microglia Interventions should be tailored to each cell type, suggesting that cell transplantation is necessary to mitigate neuron deficiency
The different types of available stem cells are vast, and as such, so are their applications in neurogenetics For example, induced pluripotent stem cells utilize transcription factors to promote specific neuronal type growth through the overexpression of particular genes, such as NeuroD1, Ascl1, Dlx2, Neurog2, Sox2, and etc After the transcription factors bind to the gene, they activate the neuronal genes in the cell and begin the production of neuronal factors and proteins to nurture the growth of the desired cell
Considering the variety of stem cell interventions–mesenchymal, endogenous, and embryonic stem cells –an effective stem cell therapy is primed to revolutionize regenerative medicine for TBIs Neural stem cells (NSCs) and Mesenchymal stem cells (MSCs)–cultivated from various tissues such as umbilical cord, bone marrow, and adipose tissue–have delivered promising results in vivo via mouse models [5]
restructure tissue [8]. Alternatively, through neurotrophic molecules, bone marrow MSCs promote angiogenesis and prohibit apoptosis. They also can inhibit neuroinflammation through the differentiation of lymphocytes, while enhancing learning and memory.
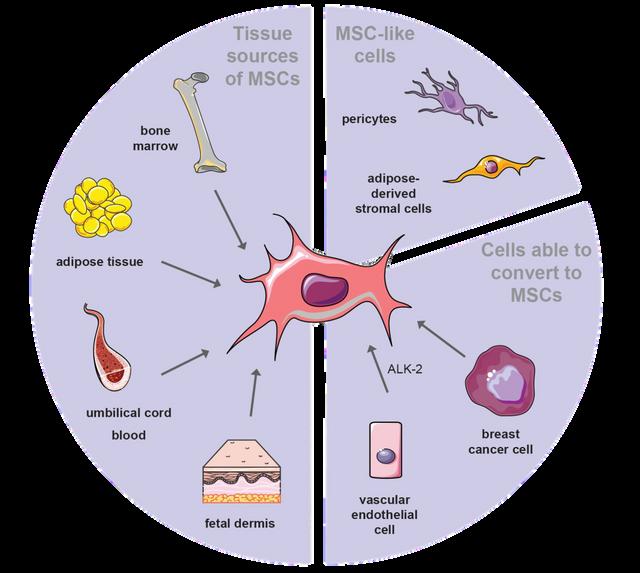
Previous studies demonstrated that endogenous NSCs can induce neuron cell proliferation and effective reintegration of neurons into neural networks with newly matured neurons. Embryonic stem cells can be transplanted into the cerebral tissue and stimulate neurogenesis/neural repair at the injury site, which would typically be at the hippocampus for TBIs [9]. Similar studies were conducted with exogenous and adult neural cells, which produced functional neurons that were able to participate in presynaptic and postsynaptic transmissions–receiving and sending both chemical and electrical signals [10]
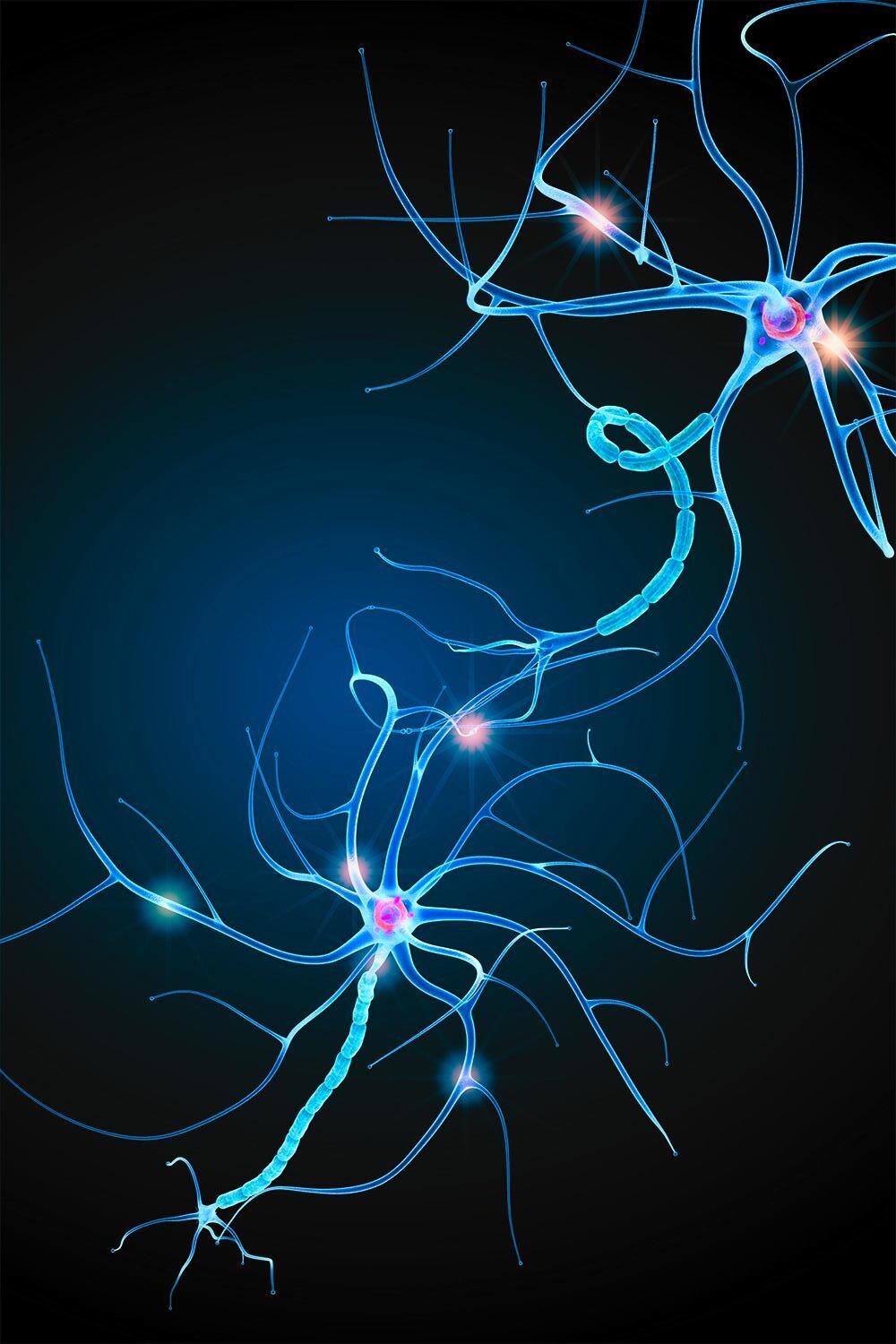
Limitations of Stem Cells
In each study, the stem cells were able to proliferate at the injury site and create functional nerve tissue. NSCs, for example, possess the ability to induce repair through the release of neurotrophic factors, cytokines, and other necessary molecules innate to the desired cell type at the injection site. This enables the tissue to retain its previously functional network, synaptic plasticity, and overall structure [6] MSCs can convert cell lineages into astrocytes or neurons at the injury site, and depending on the starting stem cell, different outcomes can be observed Adipose tissue MSCs improved the motor activity in the animal model [7], while umbilical cord MSCs promoted neural repair by influencing microglia/macrophages to
Conclusion
One major limitation of therapeutic interventions is the cost. Stem cells require high maintenance, resulting in a cost-inefficient treatment method. A large sum of the costs go to treatment clinical trials, administration of the cells during diagnosis, and observation post-treatment administration [11]. Depending on the type of stem cell therapy, costs can range from $2,000 - $100,000, varying based on the type of stem cell, delivery of cells, total number of cells necessary, quality of cells, and source of cells [12]. Mesenchymal stem cells can range from $15,000 to $40,000, for example. The multifactorial determination for the cost of stem cells accumulates larger financial responsibilities for patients and potentially renders the therapy exclusive to the wealthy [13]. Streamlining the maintenance of stem cells through future innovation could propel the creation of personalized medicine. Furthermore, delivery of the medicine, dosage, and safety concerns must be addressed prior to the introduction of stem cell technology, leaving a distant and ambiguous timeline for integration. Overall, neurotrauma persists within communities with increasing prevalence, yet it remains underrecognized. Considering the grave repercussions and expensive treatment, awareness of neurotrauma within everyday life and heavy-contact sports proves pivotal for resolving the silent epidemic ailing the United States. In light of the advances within stem cell technology, we’ve witnessed the implementation of stem cells for various diseases. Specifically, stem cells promote neuron regeneration and possess various methods of delivery to the injury site, such as transcriptional factors and injections. Stem cells provide opportunity to develop and revolutionize personalized medicine for TBIs through variation of sources for stem cells, enabling tailored stem cell intervention to adapt for specific cell type deficiencies.
WRITER: JASKEERAT GUJRAL
EDITOR: AUSTIN POTHIKAMJORN
BRAINLAB: ADVANCEMENTS INROBOTIC NEUROSURGERY
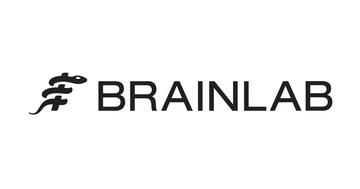
DESIGNER: JOSH MUKHERJEE
INTRODUCTION
Over the past few decades, the field of neurosurgery has made significant strides in improving surgical procedures, tools, and patient outcomes In particular, innovation has bloomed in the field of robotics and intraoperative navigation technology, which allows for enhanced surgical precision and accuracy One company spearheading this campaign is BrainLab, a German medical company based in Munich, Germany, that is working alongside neurosurgeons to develop robots that assist with cranial and spine surgery.
BENEFITS OF BRAINLAB TECHNOLOGY
BrainLab’s technology consists of highly-advanced software, mobile robotic imaging machine, pointer, drill guide, and calibration matrix, which enables surgeons to plan and execute complex surgical procedures with greater accuracy and precision The main purpose of this technology is to provide real time, accurate navigation with updated 3D scans For example, instead of performing a 2D fluoroscopy scan after the surgery, the surgeon could work in real-time and ensure the screws are in place while still in the operating room Thus, there are no delays and unnecessary revision surgeries With the help of BrainLab’s technology, surgeons can now avoid the need to take multiple images to access the correct corridor and multiple 2D fluoroscopic scans Instead, they can rely on only two arrowhead shots, which significantly reduces radiation exposure Furthermore, the technology facilitates greater precision and accuracy For example, for a spinal surgery that requires screws, the software allows the surgeon to properly plan their trajectory and evaluate appropriate dimensions The use of intraoperative planning and interbody navigation also allows the surgeon to navigate around crucial anatomical landmarks using 2D and 3D scans The technology also consists of a robotic imaging machine, which is revolutionary for multiple reasons: wireless control, greater physical working space for surgeons, automatic image registration, and larger field of view There is a special feature that allows for 3D navigation, providing an in-depth view for the surgeon while operating in narrow corridors of the operating site
Nameof Instrument Purpose
Probe (“Lenke”Probe) Used to makeacylindricalpilotholeinthe pedicle;cutsthroughboneeasily.
Possesses acentralchannelthroughwhicha metalneedle passestocutthroughbone;which is then replacedbyak-wiretoguidefollowing cannulatedinstruments;usedcommonlyin kyphoplasty/vertebroplasty
FIGURE
Drill Guide
Used commonlyincervicalcases;allowsfor navigationduringsurgery; hasa mechanism thatprevents surgeons fromdrillingtoodeep.
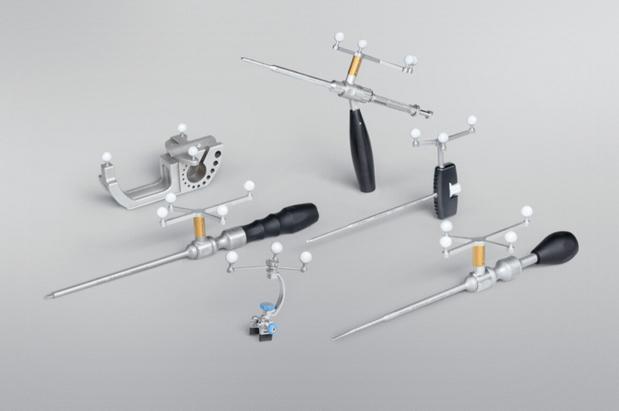
CustomReference Allows forvariety of instrumentstobenavigated
Awl Allows forperforation of the corticalboneshell.
CalibrationMatrix Allows forcalibrationofinstruments;ensures accuracyandprecision.
JamshidiNeedleAPPLICATION TO SPINAL AND CRANIAL SURGERY
In its application to spine surgery, this technology can be used to treat deformity, tumor resection, and degenerative conditions, such as scoliosis, spondylolisthesis, disc herniations, or acute spinal trauma
In regards to cranial surgery, BrainLab is utilized for craniotomies, tumor resections, and other complex cases that are difficult to access without specialized equipment
INTEGRATION OF BRAINLAB DURING NEUROSURGERY: A TECHNICAL OVERVIEW
The setup for a spinal surgery using BrainLab follows strategic calibration and precision According to a clinical specialist from BrainLab, he describes the overall process to be very straightforward The surgeon uses the calibration matrix and navigated instrument, pointing both towards the infrared camera Reference markers, which reflect infrared light back toward the camera, are utilized and cameras will recognize patterns of the markers The camera is programmed to recognize certain spatial arrangements and patterns and associate them with pre-calibrated instruments Calibration is performed by inserting the instrument into the matrix The surgeon also picks specific points in space allowing for calibration verification to take place An incision is made at the appropriate spot and the patient reference array is inserted through the fascia and clamped to the spinous process with a clamp around the bony anatomy The array is draped to ensure sterility and moved to obtain an intraoperative CT scan To protect the team from radiation, it is maneuverable from outside the OR These images are automatically transferred to the navigation system, and registered to the patient The array is moved away from the surgical field into the rest position, and registration accuracy is double checked The reference array is the mo-cap suit with the markers, but instead of a suit the reference array is a diamond structure that the camera will cross reference from and line it up 1-to-1 with the scans The camera will pick up where the markers are and where the patient is The ruler tool is used to determine the length and diameter of the screw into the spine
Once trajectory is known, a drill is used and inserted through the guide and drill is done through the drill hole and maximal depth The drill is removed and k-wire is inserted, the guide is removed and the screw is placed After successful placement of screws, k-wire is removed The change to the protocol is that k wires are not used because the navigation allows you to know your exact position This is repeated for all screws, and is verified by anterior-posterior/coronal and lateral shots are taken Navigation is used to access the disc space without extra radiation Microscope is used to further prepare for exposure of interlaminar space and facet joints Lamina, joint, and disc are removed, and the cage is placed Lastly, the final scan is done and the incision is closed Cranial surgery, on the other hand, primarily relies on software rather than instrumentation. For instance, when a surgeon takes an MRI, the software automatically segments the brain and identifies various parts of the brain. BrainLab has compiled thousands of brain scans within the system and used machine learning algorithms to automatically identify anatomical structures and organs, e.g. thalamus, ventricles, brainstem, pituitary gland, etc., that allows for automatic segmentation to occur.
PERSONAL EXPERIENCE WITH BRAINLAB
From personal experience, the technology is certainly fascinating I had the opportunity of working with representatives from BrainLab to set up the tools and practice on spine models I was able to calibrate the device and practice the screw trajectory, which is crucial for fusion and placement of screws I believe the calibration steps were clear, and the tools were easy to maneuver Although surgeons use this technology, it was easy to follow, even for an undergraduate student This demonstrates that the ease of use was a great aspect of the product
Surgeons and researchers are excited to see how technology can further advance and help the surgical world, specifically in the fields of neurosurgery and spine surgery
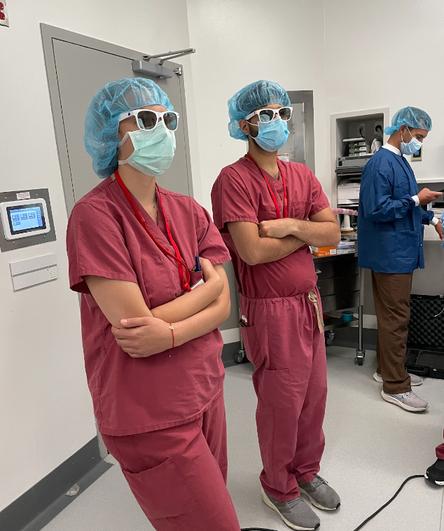
REVOLUTIONIZING MEDICAL SURGERY WITH STEERABLE NEEDLES
Writer: Ryo Lindsey
Editor: Andrew Lee
Designer: Josh Mukherjee
Millions of clinical procedures involve needles - from biopsies and thermal ablation to brachytherapy and drug delivery [1]. In the United States alone, approximately 1.6 million breast biopsies are performed annually [2]. The current needle design utilized in these highly technical and increasingly common medical procedures may lead to a multitude of complications. These complications include imprecise needle placement and tissue damage. Surgeons today are seeking less invasive methods that can target regions that are difficult to reach manually One solution is the innovative steerable needle technology, which enables procedures to be carried out faster, easier, and more precisely The applications of the technology range from minimizing extensive tissue damage during invasive surgeries to increasing the efficiency of coagulation during ablation procedures
Steerable needles are designed to be inserted into the body via a small incision and then navigated to the target area. One such design was developed by a team at the Vanderbilt Medical Engineering and Discovery Lab, which features a flexure-based tip allowing the operator to steer the needle in straight and curved paths [4]. The design consists of three nitinol wires composing the needle shaft, a beveled tip for insertion, and a flexure joint for arcing motions [5]. When the needle is inserted into the tissue, the bevel tip allows the needle to bend at the flexure joint and behave like a kinked bevel tip needle [6]. The complex components of the flexure tip needle are significantly different from the traditional rigid needles. While the ability to maintain a straight path is desirable, if the needle will miss the intended target, reinserting the needle is necessary to alter the path. Taking out a needle and reinserting it into the body is time consuming and can result in more tissue damage For example, this problem becomes apparent in prostate cancer surgery when radioactive seeds are placed precisely through the use of needles A patent for a computer-aided needle design notes that if the needle is tangential to the prostate capsule wall upon penetration, it will lead to deflection and deformation of the needle The deformation can lead to further damage of the tissue surrounding the prostate which can lead to follow-up surgeries Steerable needles, on the other hand, allow for more accurate localization to tumor sites [7] Some steerable needles are developed so that they can follow the optimal 3 dimensional curvilinear path to avoid critical anatomical structures [8] This increased fluidity in mobility helps to minimize trauma to the surrounding tissue and reduce the risk of complications.
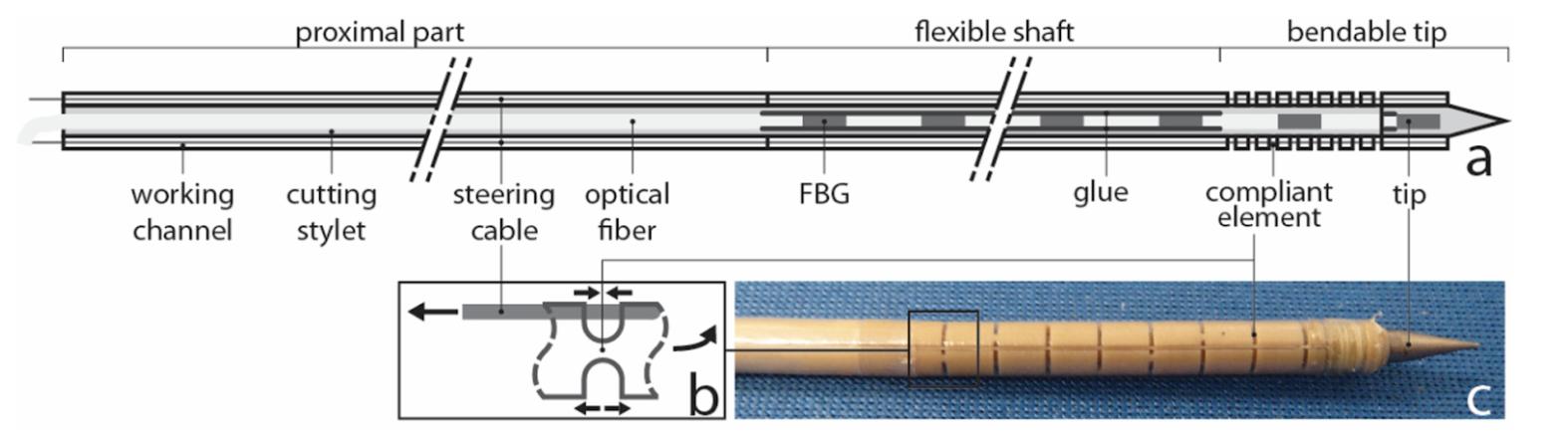
Major advancements in the steerable needle technology have been developed by Mark Yim, Asa Whitney Professor of Mechanical Engineering and Applied Mechanics, and his colleagues at the University of Pennsylvania With over 250 patents, including one detailing a novel approach to the technology called “Sharp Turning Steerable Needle,” [9] he is one pioneer at the forefront of the field
The 2021-2022 Y Prize competition, a prestigious competition held yearly at the University of Pennsylvania based on entrepreneurship and innovation, made one of its headline technologies the steerable needle. Teams of undergraduate students researched and pitched ideas on how to best use the technology Dr. Yim developed. Some groups proposed using the steerable needle to improve brain stimulation therapy, perform chemotherapy injections, and reduce pain in breast biopsies [10]. However, the grand prize went to Ossum Technologies, a company that proposed the use of the steerable needle technology for orthopedic surgery procedures Specifically, the team’s vision involved increasing the safety of cerclage wire use in fracture fixation The procedure involves the wrapping of a cerclage wire around the fractured bone to repair the damage and provide effective fixation The cables would enable sharp steering of the needle tip and the cerclage wire will have the ability to attach onto the bone tightly increasing precision A soft tissuefriendly 3mm needle would be attached to a standard surgical tool grip with blunt edges to minimize neurovascular damage Due to the needle’s added flexibility, it will easily be able to maneuver around complex bone structures and allow for a minimally invasive procedure [11].
Ossum Technologies is just one of many companies around the world that are researching steerable needle technology. This highly versatile technology has taken the world by storm and its medical applications are endless. With further development of steerable technology in conjunction with artificial intelligence, there is no doubt that it can be applied to other complex medical procedures. Many notable universities including Stanford, Washington State University, and Pennsylvania State University have laboratories set up to further develop the technology. Furthermore, in a recent article published by the Institute of Electrical and Electronics Engineers Journal, the potential application of steerable needles with artificial intelligence was explored In this study, scientists developed a novel approach to optimize lung cancer procedures with search trees and algorithms to better analyze the path of the steerable needle in the body [13] These path planning algorithms relied on models of needle-tissue interactions for accurate needle alignment and control [14] Steerable needles are a promising technology that can improve the safety and effectiveness of highly complex medical procedures While future research is warranted to develop steerable needles further, they have the potential to completely revolutionize the way invasive surgeries are performed Steerable needles will likely become a standard medical instrument in the near future
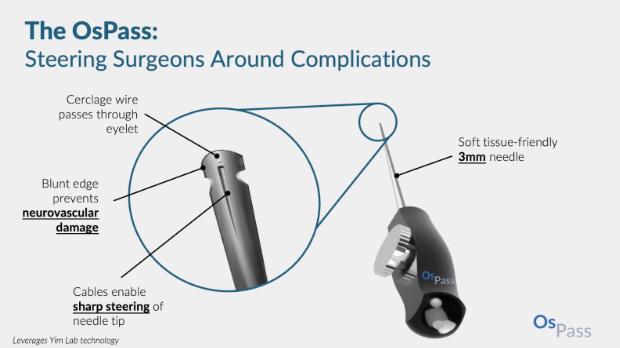
LeadlessPacemakers
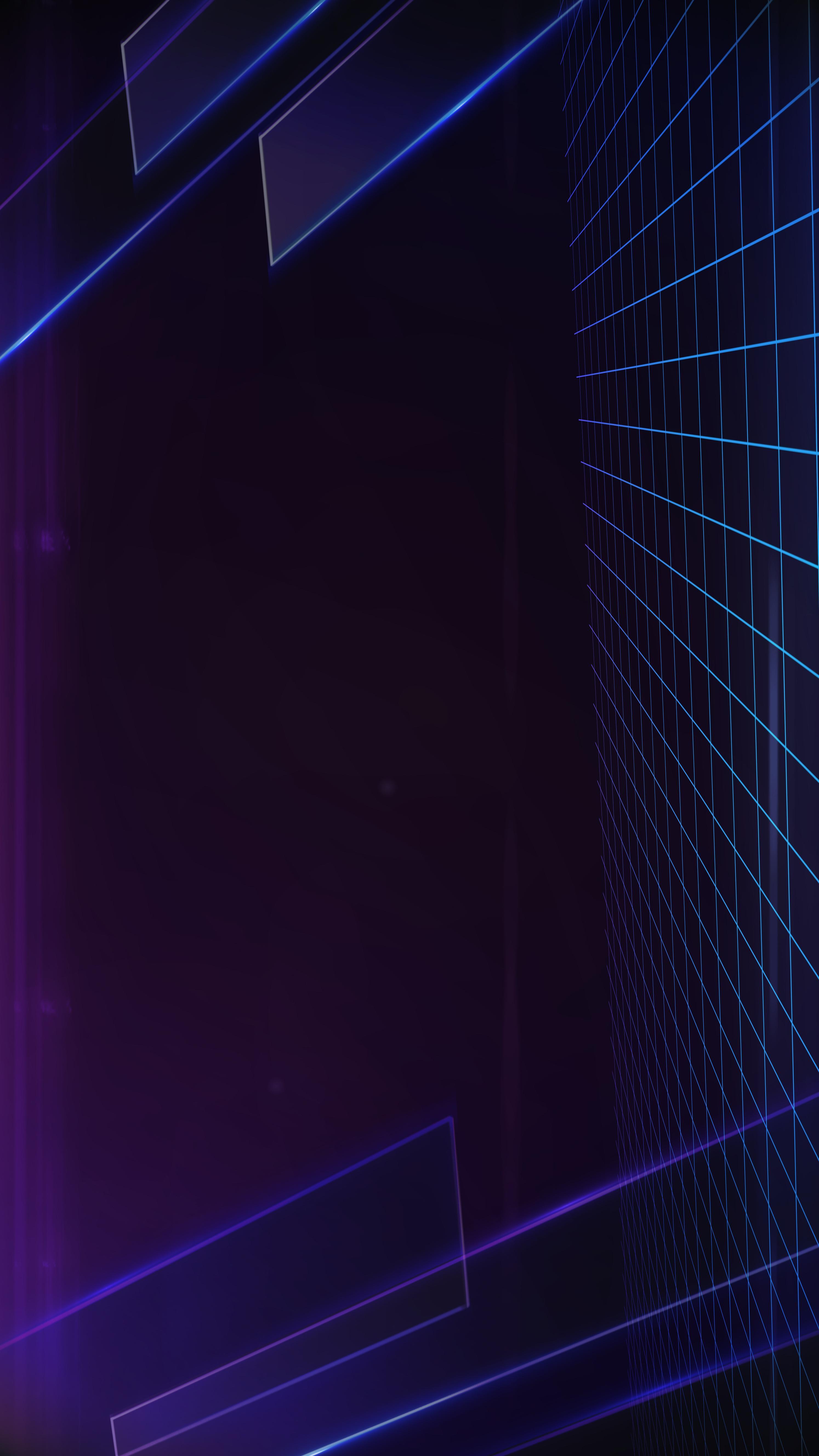
SettingthePacefortheFutureofElectrophysiology
WrittenbyAshilSrivastava EditedbyJessicaWu DesignedbyJuliaGerbinoFew inventions have been more critical to the field of cardiac electrophysiology than pacemakers Created in the 1950s by Dr William Chardack, Dr Andrew Gage, and engineer Wilson Greatbatch [1], pacemakers have elongated the lives of millions by correcting irregularities in heartbeat rhythms [2]. Since the invention of the original pacemaker decades ago, the device has undergone numerous innovative developments, the most recent being the leadless pacemaker.
A pacemaker works by sending electrical signals to the heart to stimulate the ventricles When an irregular heartbeat is detected, the pacemaker brings the heart’s rhythm back to an optimal rate Most patients who need pacemakers suffer from bradycardia, a condition in which the heart doesn’t beat fast enough. Bradycardia can be caused by heart attacks and heart transplants if the body struggles to adjust to the new heart. Another condition treated by pacemakers is Tachy-Brady Syndrome, which makes the heart beat abnormally fast or abnormally slow at varying times [4]. There are also patients using pacemakers who have a condition called sudden cardiac arrest, where their heartbeat stops momentarily
Conventional pacemakers contain three main components The pulse generator, inserted just below the collarbone, is a small, battery-powered metal case that regulates the electrical signals sent to the heart [5]. Multiple leads, or insulated wires, connect the generator to the heart’s chambers. Each lead ends in an electrode that detects the heartbeat and delivers electrical impulses to the heart [6]. When the heart rate falls under a certain threshold, the pacemaker is programmed to kick in, and the heart responds accordingly.
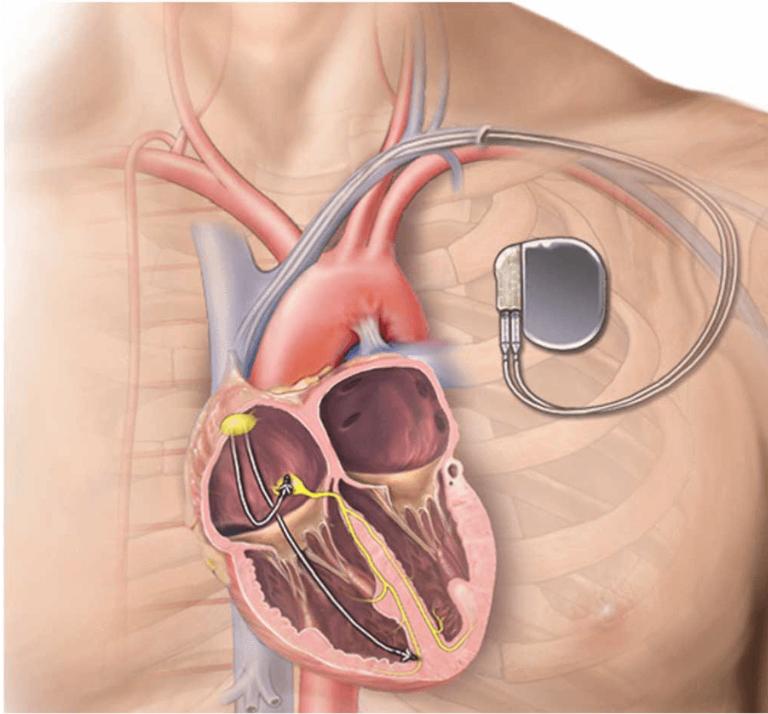
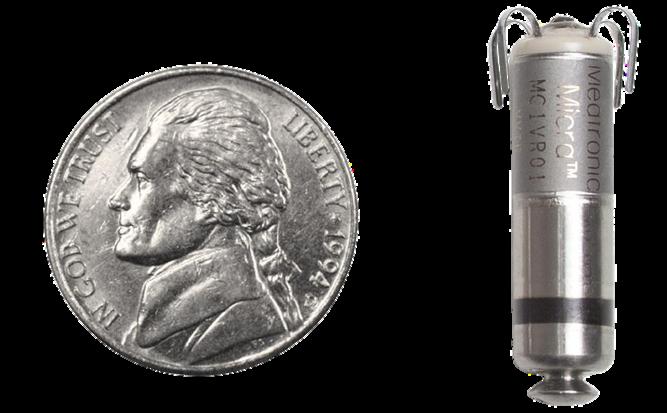
The leadless pacemaker is a more efficient iteration of its predecessor Inserted in the right ventricle of the heart, the leadless pacemaker is tubular-shaped and 90% smaller than a traditional pacemaker. While a leadless pacemaker functions similarly, it has no external wires and serves as its own generator [8]. This eliminates many of the potential problems associated with the extra hardware, reducing almost 50% of vascular complications and device malfunctions [9]. Aesthetically, the leadless pacemaker is more discreet than a normal pacemaker because it doesn’t form a bump under the skin [10] The leadless pacemaker has similar, if not better, battery efficiency than a normal pacemaker
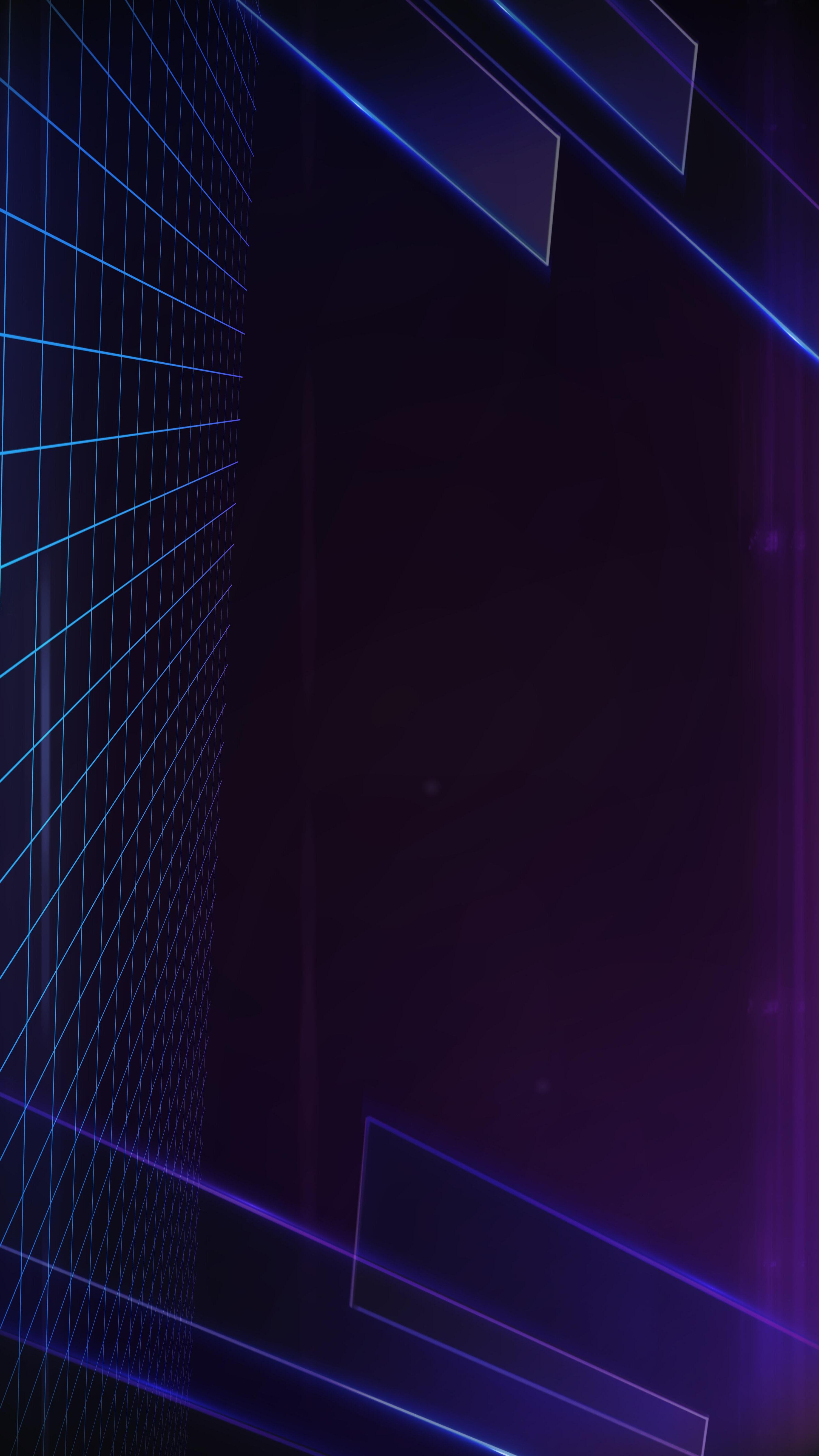
Leadless pacemakers also offer benefits from a surgical perspective Implantation of the device is performed via a catheter, an extremely narrow tube that is inserted in the femoral vein and guided up to the heart [5] The catheter is removed once the pacemaker is properly secured in the right ventricle and attached to the heart wall. The whole process takes about half an hour with relatively low risks such as internal bleeding [5].
To date, two companies have manufactured a leadless pacemaker One is the Micra, made by Medtronic, and the other is the Nanostim from St Jude Medical The FDA has approved only the Micra for use [11] The Micra costs around $10,000, which is significantly more than the $2,500 cost for a normal pacemaker [12] These prices do not factor into account other fees, such as surgical costs and imaging.
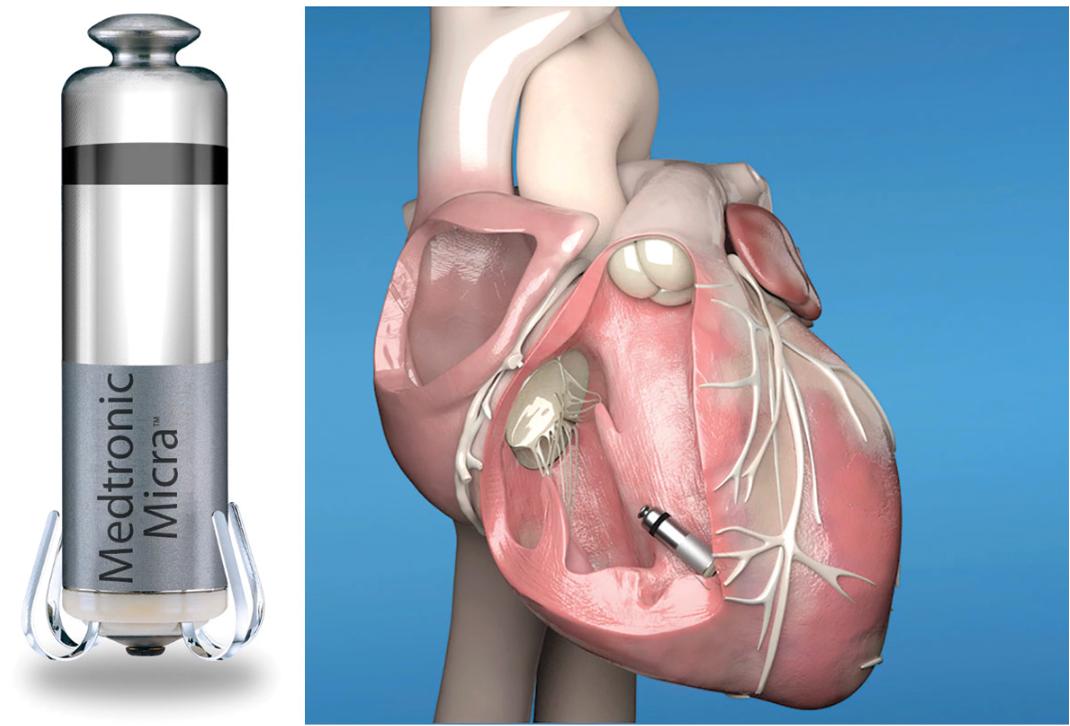
The Nanostim had many reliability issues, including premature battery failure. Eventually, Abbott Laboratories bought St. Jude, and they are currently working on developing their own version of the leadless pacemaker called the Aveir VR. The Aveir VR will have a battery life double that of its competitors and will make it easier to locate the right area for placement before surgery [13]
The medical giant Boston Scientific will also be entering the pacemaker scene with hardware of their own to combat tachycardia, which is a condition in which the heart beats alarmingly fast [6]
It seems that, as of now, leadless pacemakers are the future of pacemaker technology The Micra was only developed in 2016, making this technology relatively new and cutting edge. However, more innovations are on the horizon. The first leadless dual chamber pacemaker, which functions in the right ventricle and atrium, was implanted successfully in the Czech Republic [14]. Scientists are currently working on creating a leadless biventricular pacemaker, which would function in both the right and left ventricles [4]. Battery life is always going to be an area of improvement to lengthen pacemaker efficacy The future of pacemaker technology is promising with the next generation slated to be the most effective and efficient
Organ on a Chip
The Future of Biomedical Research
Written by Alex Gerlach Edited by Ashrit Challa Designed by Ashley Toresembles the natural environment of the organ. The cells are then fed with nutrients and oxygen through tiny channels, simulating the flow of blood and other fluids in the human body. This specific engineering enables the cells to receive nutrients and oxygen at the same rate as in the human body, allowing the cells to remain viable and functional for an extended period. The construction of a given Organ-on-a-chip requires sophisticated technology, implementing microfabrication, 3D printing, and microfluidics. The microfabrication allows for the precise control of the dimensions and shape of the device, while 3D printing enables the creation of the complex structures that constitute it with high accuracy. Finally, microfluidics work gives rise to the precise control of the flow of fluids, enabling the simulation of fluid flow as it occurs in the human body. The summation of these engineered features results in an anatomically realistic device that bears immense potential in the treatment of those with malfunctioning organs [2, 3].
APPLICATIONS AND ADVANTAGES
INTRODUCTION
There has been a longstanding quest to create more innovative, efficient, and ethical methods of drug development, personalized medicine, and testing. While the concept of stem cells and artificially synthesized implants have been around for a while, advancements in medical research and technology have recently led to the development of an even more innovative biomedical device known as an Organ-on-a-chip. This device, pioneered in 2010 [1] and exponentially evolving since then, distinguishes itself in its designed ability to mimic both the structure and function of human organs, leading to previously unconceived advances. Although many exist, three
distinct ways that this innovation holds promise will be discussed: 1) drug development, 2) personalized medicine, and 3) sustainable future medicine.
WHAT IS AN ORGAN ON A CHIP?
An Organ-on-a-chip is a small microfluidic device that contains living cells arranged in a threedimensional structure to optimize mimicry of a human biological organ. The cells involved are arranged on a thin, flexible membrane that is coated with extracellular matrix proteins to create a microenvironment that
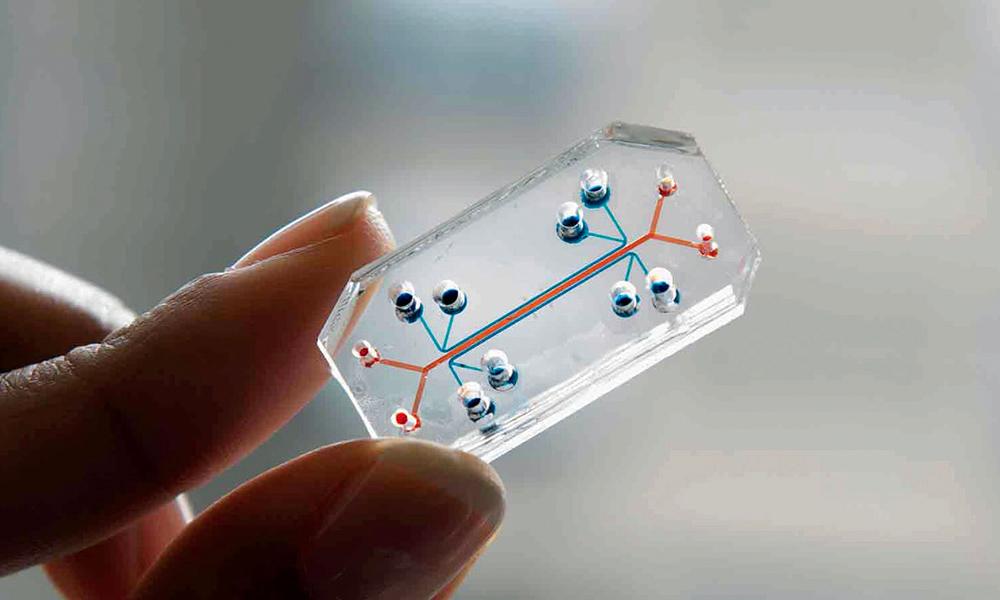
While still in its nascent stages of progress, researchers are already using the Organ-on-a-chip model to study various human organs, including the lung, heart, liver, kidney, and intestines. The results have shown efficacious progress made in drug development, personalized medicine, and sustainable future medicine.
Drug Development. The model boasts an unparalleled advantage in the field of research by closely emulating human physiological conditions, a feat not previously achievable through traditional cell culture or animal models. This leads to enhanced precision and relevance when testing drugs inside the body, yielding results that mirror real-world scenarios more accurately. One of the monumental components of drug development is testing for toxicity, which the Organ-on-a-chip technology also shows promise in advancing. Traditional animal testing for toxicity has limitations due to the inherent biological differences between animals and humans that result in discrepancies in drug efficacy and side
DID YOU KNOW?
effects. Moreover, the traditional in vitro methods for testing toxicity do not accurately mimic the complex physiological environment of a living organism, which leads to even less reliable results [4,5].
Organ-on-a-chip technology provides an exciting solution to these challenges by offering a more realistic representation of human physiology in a lab setting. The incorporation of human cells into these chips allows for a more accurate representation of how toxic substances affect human organs. Researchers can also customize the chips to mimic specific organs, such as the liver, which detoxifies many substances in the body. With this technology, researchers can more accurately study the effects of toxic substances on human organs and develop safer, more effective drugs. And perhaps even more importantly, the organ-on-a-chip innovation minimizes reliance on both costly and time-consuming animal testing, testing that is also often deemed ethically controversial [6, 7].
Personalized Medicine. Organ-on-a-chip technology is also being used for personalized medicine. By specifically targeting a patient's own cells, researchers can create a personalized organ-on-a-chip to study how a patient's body would respond to a particular drug or treatment. This not only allows for more accurate and effective treatments, but it simultaneously reduces the risk of adverse reactions and side effects. Another critical component of personalizing medical care is examining the ways in which diseases are modeled. Researchers can use these chips to create disease models that closely mimic human physiology, which allows for a better understanding of disease progression and potential treatments. For example, organ-on-a-chip models of the lung have been used to study pulmonary diseases such as asthma and chronic obstructive pulmonary disease (COPD). By studying the progression of these diseases in a more accurate model, researchers can replace generalized treatment plans of the past with approaches that hone in on much more specific aspects of the disease [8].
Sustainable Future Medicine. The benefits posed by this device also expand into the realm of considering how medicine might look in the future. One way in which this manifests is through the field of space travel medicine. Long-term space travel presents numerous challenges to human
physiology, including muscle and bone loss, cardiovascular dysfunction, and radiation exposure. However, by using organ-on-a-chip technology, researchers can simulate the effects of long-term space travel on human organs and consequently develop strategies to mitigate these effects. This technology could also be used to develop more effective treatments for astronauts who experience health issues during space travel. Regenerative medicine is another term that often comes to mind when considering sustainable medical practices of the future. Organs-on-a-chip show potential here by creating an environment that mimics the natural physiology of the body. This allows researchers to develop and test new therapies for tissue repair and regeneration; for example, researchers have used organ-on-a-chip technology to study the regeneration of muscle tissue after injury. With further evolution, this technology could potentially lead to the generation of personalized therapies for patients with tissue damage or disease [9, 10].
LIMITATIONS
Despite its many advantages and potential applications, Organ-on-a-chip technology also has some limitations. Due to its still early nature regarding development, there is still much to be learned about how to create accurate models of human organs. It also demands complex and expensive technology, requiring specialized equipment and expertise that may offset some of the aforementioned benefits in research. The production of these nuanced chips is currently limited to small numbers, and so the large-scale drug testing promised might be in reality much more speculative than possible. The technology is stifled even further by its limited ability to mimic the complex interactions between different organs in the human body. Many diseases and drug interactions involve this exact kind of interplay between multiple organs, and thus questions can be posed regarding the comprehensiveness of the device [11].
CONCLUSIONS
DID YOU KNOW?
The technology of Organ-on-a-chip shows an area full of promise for research that could change fields not just limited to drug development and personalized medicine. The distinct advantages include a more realistic representation in lab settings that simultaneously reduces inefficiencies and immoral tests, but there are also challenges that must be faced in financial, scaling, and comprehensiveness aspects. Despite these, however, the Organ-on-a-chip renders itself a worthwhile device to continue exploring.
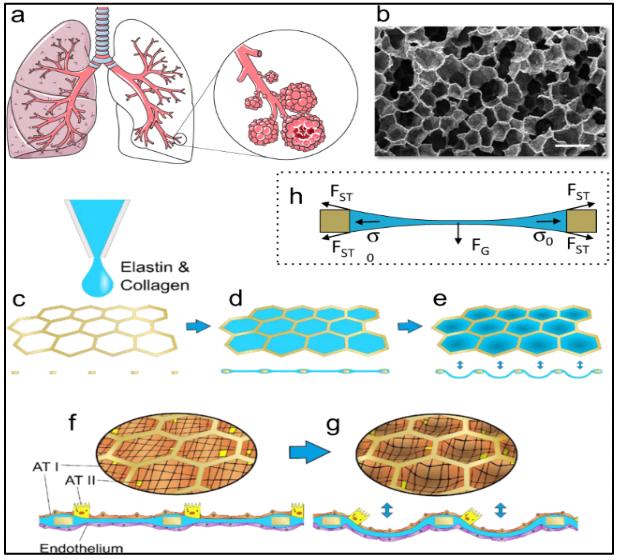
Stereoelectroencephalography: A Breakthrough in Mapping Neural Responses and Sensory Perception
Stereoelectroencephalography (SEEG) is a novel and innovative brain imaging technology that has revolutionized how we study and treat the human brain. Initially popularized in France during the 1950s for patients with refractory focal epilepsy, SEEG has since advanced in methodology due to improved computer technologies and implantation devices SEEG is unique as a minimally invasive surgical procedure that begins in an operating room with a patient under anesthesia Neurosurgeons place thin electrodes directly into targeted brain areas, and electrodes can then record the electrical activity in the brain SEEG is commonly utilized for patients with difficult-totreat seizures, where monitoring can pinpoint the precise source of seizure activity After this location is determined, surgeons can attempt to remove that section of the brain to eliminate or reduce the number and severity of seizures [2]. Furthermore, recent research has advanced the applications of SEEG by expanding to areas including touch stimulation and brain mapping [3,4]. In this paper, we will examine the development of SEEG, exploring its progression from its early roots in epilepsy treatment to its current status as a versatile and adaptable tool in the broad field of neuro-engineering. With its proven track record of success, SEEG research stands at the forefront of cutting-edge research and holds great promise for uncovering new insights into the human brain
Epilepsy is a persistent brain disorder that affects an estimated 50 million people globally, characterized by sudden and unpredictable recurring seizures While some seizures rarely cause significant problems for people besides common injuries such as cuts, successive untreated seizures can lead to more severe
consequences, such as permanent injury or death.
Sudden unexpected death in epilepsy (SUDEP) is the most common disease-related cause of death in people with epilepsy, leading to the importance of seeking treatment from epilepsy specialists [5] Excessive electrical discharges in a group of brain cells induce seizures [6] Antiepileptic drugs (AEDs) are the most commonly utilized treatment for epilepsy and successfully control seizures in around 7 out of 10 people [7] However, for patients with drug-resistant epilepsy, surgical intervention is utilized as an effective treatment alternative [8]
The history of SEEG began with its founder, Jean Talairach (1911-2007) Together with neurosurgeon Jean Bancaund, they used SEEG to implant deep electrodes in patients with epilepsy in Paris. By using both ventriculography and angiography data, their techniques quickly became adopted by others across France and the world, establishing SEEG as a widely recognized technique for diagnosing and treating epilepsy. In Madrid, neurosurgeons developed simultaneous video and EEG recordings, creating the foundation for modern video EEG monitoring. Soon after, Italy's first center for SEEG and the treatment of epilepsy opened Around the same time, SEEG was found successful in a study in Canada in 70 patients
patients with epilepsy For many years, the complexity and duration of the implementation procedure severely limited SEEG However, recent advancements in stereotactic implantation and robot-assisted surgery have expanded the usage of SEEG in surgical treatments of epilepsy[9]
The utilization of SEEG as a diagnostic tool for patients with drug-resistant epilepsy has undergone significant advancement over the years. Modern neuroimaging studies and digital EEG have allowed for the improvement of locating and accessing the epileptic network. The SEEG method has been favored over alternative methods, such as subdural EEG (SDEEG), due to its capability to cover extensive areas of bilateral hemispheres and accurate sampling in deep brain structures and the sulcal regions. Recent meta-analysis studies indicate that complications associated with SEEG are significantly less than SDEEG, in part because of easier electrode removal, which in turn allowed for sufficient time in data analysis and treatment planning for patients and physicians [10] The overall rate of complications was lower with SEEG implantation (1 3%) as compared to SDE (3 5%), including decreased hemorrhagic and infectious complications In conjunction with the rate of the neurologic deficit being more favorable with SEEG, SEEG appears to have a more favorable safety profile overall for invasive monitoring [11].
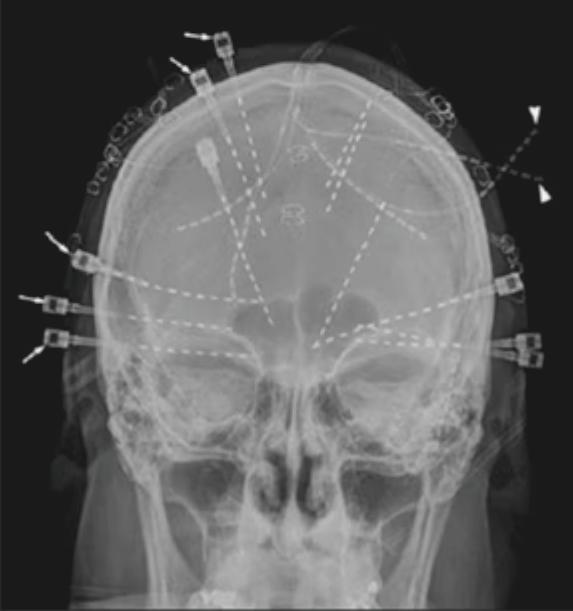
Building upon its established efficacy of SEEG as a traditional tool used for drug-resistant epilepsy, SEEG has now been explored for its potential to evoke a sense of touch in patients who have lost it due to paralysis, neuropathy, and other illnesses and injuries. A recent study by researchers at The Feinstein Institutes for Medical Research highlights the use of SEEG implants in restoring touch, improving the lives of patients who can now perform everyday activities, and benefiting their overall emotional well-being This current study goes beyond past research that has utilized braincomputer interface technology to stimulate
areas of the brain and restore some sensation to the hand With its ability to access more obscure sensory regions of the brain, SEEG can access sulcal grooves, evoking much more precise sensory percepts in the fingertips. In addition, the electrodes provided helpful somatosensory feedback for dexterous hand movements, invoking tactile sensations. The study’s success in targeting fingertip regions and mapping neural responses during the intraoperative process demonstrates SEEG as a potential solution for restoring touch [12].
Despite the benefits of SEEG, SEEG still presents limitations in specific capabilities The workflow of SEEG is intricate, thus calling for the precise imaging of the cerebral vasculature and several other regions that may not be feasible by many clinics Additionally, SEEG also underperforms compared to SDEEG in mapping atypical representations of the eloquent cortex [13] Nevertheless, the potential of SEEG in advancing our understanding of the brain and developing treatments for neurological conditions cannot be ignored This research progress provides hope for better outcomes and further work in improving patients’ quality of life affected by neurological conditions.
Alzheimer’s Disease Diagnosis Using Deep Learning
Writer: Sunny Wang
Editor: Jessica Guo
Designer: Oscar Capraro
Alzheimer’s disease (AD) is a progressive disease characterized by the loss of cognitive ability and is the sixth leading cause of death in the United States. AD is typically classified and diagnosed based on a variety of factors, including cognitive and laboratory tests.
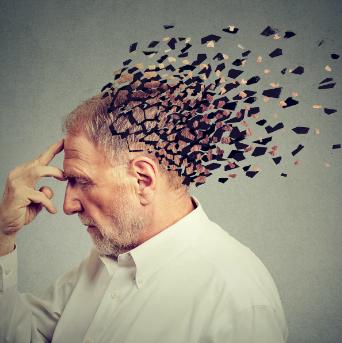
According to a study conducted by Beach et. al., the overall clinical diagnosis accuracy was 77% with a low true negative rate [1]. This is far from perfect, increasing the demand for a computer-assisted tool to reinforce physicians' diagnoses.
As AD causes the breakdown and death of neurons, these changes in brain mass can be observed through technology such as magnetic resonance imaging (MRI). These scans are suitable for computer vision and deep learning algorithms, such as the convolutional neural network (CNN), which has achieved impressive results in classification. Diagnosis of AD using machine learning can serve as a powerful tool for physicians, supplying an additional metric for diagnosis.
CNNs typically require large datasets to perform effectively. However, medical data is often scarce and limited in size, largely due to the high standards of consistency and organization required for medical data, and the cost and time required for data collection. This raises a demand for data augmentation
techniques to improve medical machinelearning models. One recently introduced technique is the generative adversarial network (GAN), which achieves promising results in image generation [2]. Using a relatively small dataset, GANs can generate similar but original images, as opposed to image modifications used in classical data augmentation.
The use of CNNs for Alzheimer’s disease diagnosis has become more prominent in recent years. Hosseini-Asl et al. [3] used a deeply adaptive 3D convolutional neural network (DSA 3D-CNN) achieving a 94.8% accuracy in task-specific classification. Glozman et al. [4] proposed a network of 2D CNNs, applied to each of the three images extracted from each sample, which achieved a maximum of 83% accuracy. However, there have been relatively few applications of GANs in classifying AD, with none of them using it for data augmentation.
In this study, we investigated the potential for using deep learning in Alzheimer’s disease classification by creating a convolutional neural network model. We also tested the feasibility of using GANs for data augmentation, specifically using the CycleGAN architecture [4]. To do this, the steps involved data acquisition, data preprocessing, CycleGAN-based Data Augmentation, and Convolutional Neural
Network Classifier, as seen in Figure 1.
We constructed CNN models utilizing the ResNet50 architecture to diagnose Alzheimer’s disease while also addressing the problem of size limitations in medical datasets with the use of generative adversarial networks (GANs). Using the ADNI1 dataset, we demonstrated that the addition of GANs can greatly improve the classification accuracy of deep learning models for Alzheimer’s disease diagnosis. Specifically, we used CycleGAN to generate images of one class using the other, balancing the dataset and increasing its overall size. Our results show that classification accuracy improved substantially, increasing the F1 score from 0.891 to 0.951. Due to the lack of large datasets in many medical fields, the results obtained in this study can be generalized to many other medical imaging fields as well. With promising results in data augmentation, GANs have the potential to significantly improve classification tasks across a wide variety of applications.
Future work should be done to improve the model, the first of which is preprocessing. Upon inspection, the current methods of preprocessing yield some inconsistency between images, particularly in axial scans, due to subjects having slight variations in brain shape. In the future, other methods of preprocessing could be used to improve consistency across each slice taken. Software
such as statistical parametric mapping could also be applied to perform preprocessing such as gray matter segmentation and modulation to reduce variation between images. Moreover, the current model serves to classify between NC and AD samples. The intermediate stage, mild cognitive impairment (MCI), could also be incorporated into the classifier. Augmented CycleGAN could be implemented to generate images of all three classes. Lastly, diagnosis of Alzheimer’s Disease often uses both MRI scans and PET scans. In contrast to MRIs, which provide identification of abnormalities in the brain, PET scans can show areas of low metabolism, allowing for differentiation between Alzheimer’s and other types of dementia. Using a fused image with both scans in conjunction would contain more features and will likely improve classification accuracy.
In conclusion, this study has demonstrated the potential of utilizing deep learning and GANs to facilitate Alzheimer’s disease diagnosis. Even while utilizing a small dataset, the CycleGan architecture for data augmentation was able to significantly improve the classification accuracy of the CNN model. These findings suggest that GAN-based data augmentation could be a powerful tool in medical imaging fields with limited data availability. Thus, this study provides a promising foundation for future research in medical imaging analysis and improves the field of medical diagnostics.
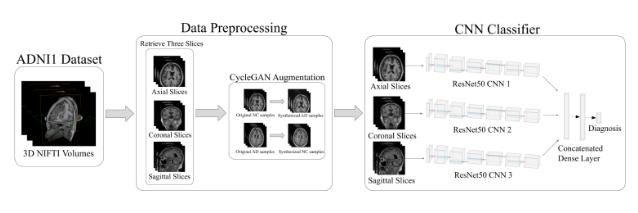
To Be Or Not To Be?:
The Curious Journey of Neuralink
Written by Neil Tangal | Edited by Hiba JamilIn 2015, Elon Musk cosigned an open letter with Steven Hawking and a multitude of other experts warning the world about the inevitability of artificial intelligence surpassing humans [1]. To prevent this, Musk envisioned merging AI with human intelligence to reach a new level and achieve a form of transhumanism [2]. A year later, Neuralink registered as a start-up and was publicly announced by Musk in a 2019 presentation and white paper [3]. The company cited its short-term goal as improving life for people with neurological disorders and eventually enhancing humans in the long term. Since then, the company has worked on its novel N1 chip, a brain-computer interface (BCI), that can transmit neural signals to outside technology In theory, this has a ton of applications, the main one being the ability to bypass spinal cord damage, which would change the lives of paralysis patients The technology is still some years from widespread adoption, but the potential is undeniable
The idea of a neural interface is not a new one Around the turn of the 20th century, Jean-Marc Cote and a host of other French artists produced a collection of paintings titled “France in the year 2000” for display at the 1900 World’s Exhibition in Paris [4]. One painting portrayed a classroom of students with head caps wired to a machine illustrating another child cranking the machine while the teacher puts textbooks into it. The roots of BCI research can be traced back to the 1920s. Hans Berger, a German scientist, was the first to record an electroencephalogram (EEG) of a human brain using electrodes on the skull By the 60s and 70s, researchers realized that the signals had to be continuously and immediately transcoded digitally
Jacques Vidal, a professor from UCLA, wrote up all the elements of a BCI and suggested that the digital signal could control external devices such as prosthetics. However, it wasn’t until the 2000s that BCI development advanced due to increased information transfer rates and user-centered design. The first users of such a device were two patients with locked-in syndrome (LIS) and amyotrophic lateral sclerosis (ALS): their implanted device enabled them to communicate non-verbally [5].
Overall, Neuralink had a solid foundation to draw upon. After purchasing the name trademark from its previous owners, Musk and a team of engineers, including Max Hodak, got off to a quick start A total of $158 million arrived through Series A and B funding in 2017 and 2019 respectively, with the overwhelming majority coming from Musk himself [6] The two rounds of funding were to establish the company, obtain a market share, and scale the opportunity. The initial idea was to develop some kind of neural lace (thin mesh capable of monitoring brain activity): Musk has pointed to Iain M. Banks’ sci-fi novel, “The Culture” as inspiration [7]. Information would be relayed to the N1 chip which would then wirelessly transmit the data to outside devices, such as a prosthetic arm. Initial product development was positive, as Neuralink received $205 million in Series C funding from no less than twelve different investors including Google Ventures, Valor Equity Partners, and Vy Capital, a UAE based firm [8]
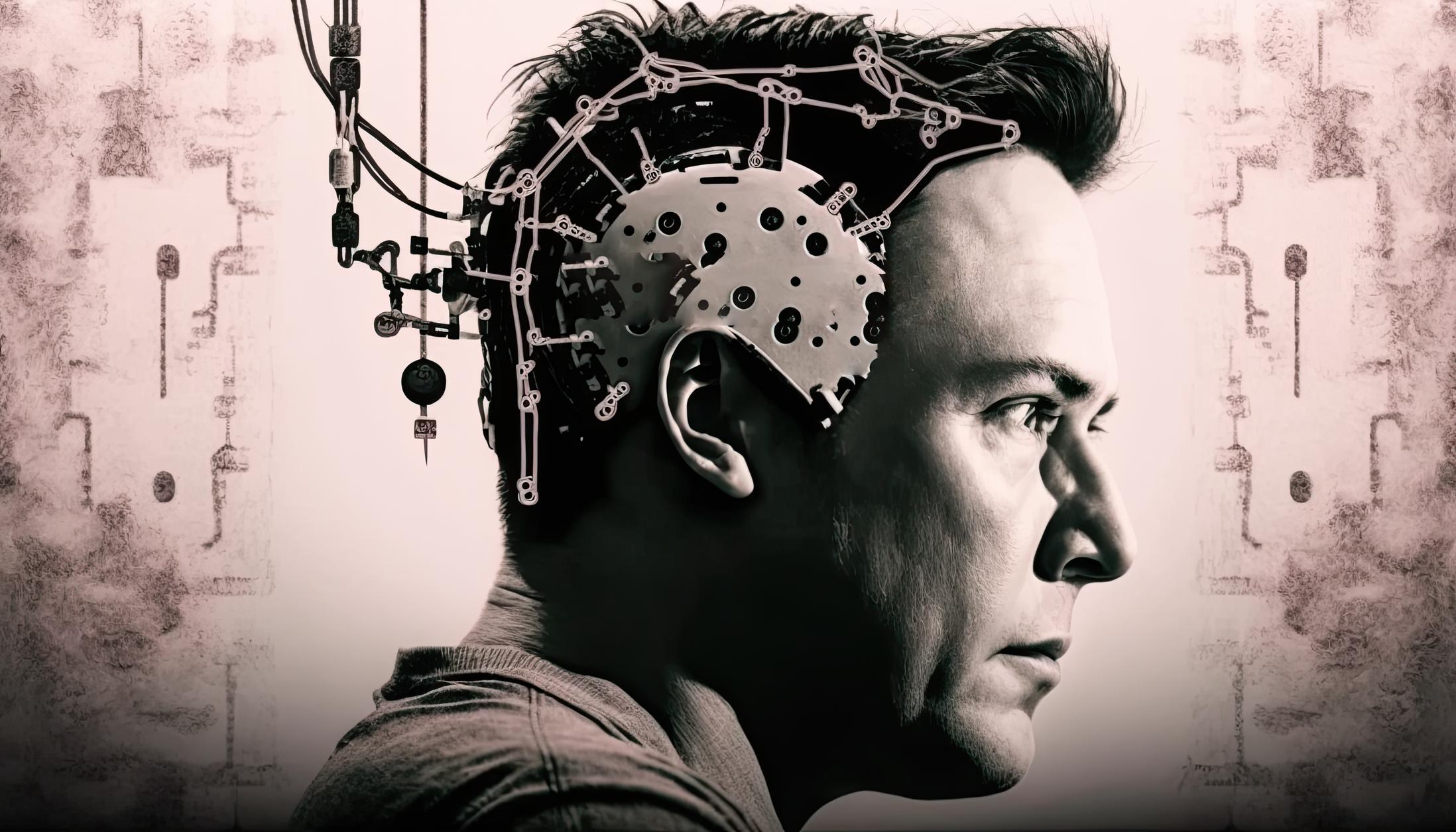
So what was it that all these investors saw potential in?
The technology itself works as follows. The human brain is constantly firing action potentials along neurons, which serve to transmit nerve impulses. Neuralink has developed an ultra-thin thread (4 - 6 μm) that can pick up signals and can be directly stitched into the brain. These threads are organized into complex arrays which enable a high-volume data transfer. To properly implant these wires, Neuralink developed a robot that can insert them in the least invasive manner possible, most importantly avoiding vasculature and bleeding The data is then relayed to the N1 chip, which will consolidate and analyze the data, and then wirelessly transmit it to an external device, such as a mobile app [9] What sets this technology apart from others is the sheer amount of channels available for data transmission. Early animal trials with rats proved successful in basic tasks. Famously, Neuralink released a video in 2021 showing a macaque monkey using a brain implant to play Pong with its mind [10]. Primarily, the company sees this technology being used to improve the lives of people with disabilities, such as restoring sight for blind people, or enabling people with severed spinal cords to walk again. Human trials have been submitted to the FDA for approval [11].
However, the project is not without its criticism The biggest concern is an ethical one How morally correct is it? Opponents of the project point to “neurohype”, postulating that Musk is exaggerating neurological innovation, as a major concern [12] If unsubstantiated, Musk’s claims could hurt innovation by forcing policymakers to make tough decisions about unproven technology. Musk has been accused many times of exaggerating his company ’ s lofty achievements as we ’ ve seen before with Tesla’s autonomous cars. Additionally, Neuralink faced a federal probe in December 2022 regarding animal welfare violations. Internal documents from the company revealed that over 1,500 animals had been killed in testing, with loads of unneeded suffering due to botched experiments and rushed procedures. Former employees have slammed Musk, criticizing his obsession with speedy progress rather than employee and animal welfare [13]
Neuralink is not unique in its ventures either Most prominently, biotech company Synchron has beat Neuralink to the punch regarding human trials: in July 2022, their BCI was implanted into a patient using a novel endovascular delivery method, which is less invasive than open brain surgery. Interestingly, Max Hodak, a cofounder of Neuralink who left the company in 2021, was found investing in Synchron [14]. Meanwhile, despite Musk promising the initiation of human trials back in 2019, the company is still waiting for FDA approval well into the start of 2023. Overall, it hasn’t been perfectly smooth sailing for Neuralink. In the rapidly evolving world of engineering medicine, Neuralink’s journey has been notable They have set forth lofty goals for its technology, which have gone through new advancements and improvements The possible applications could be life-changing, but the project is not without its critics Concerns about internal conflict at the company along with suspected animal welfare abuses have hamstrung the project compared to its competitors. However, Neuralink has massive potential, particularly in the field of prosthetics and other neurodegenerative diseases. It’s worth watching if Musk can properly realize this potential.
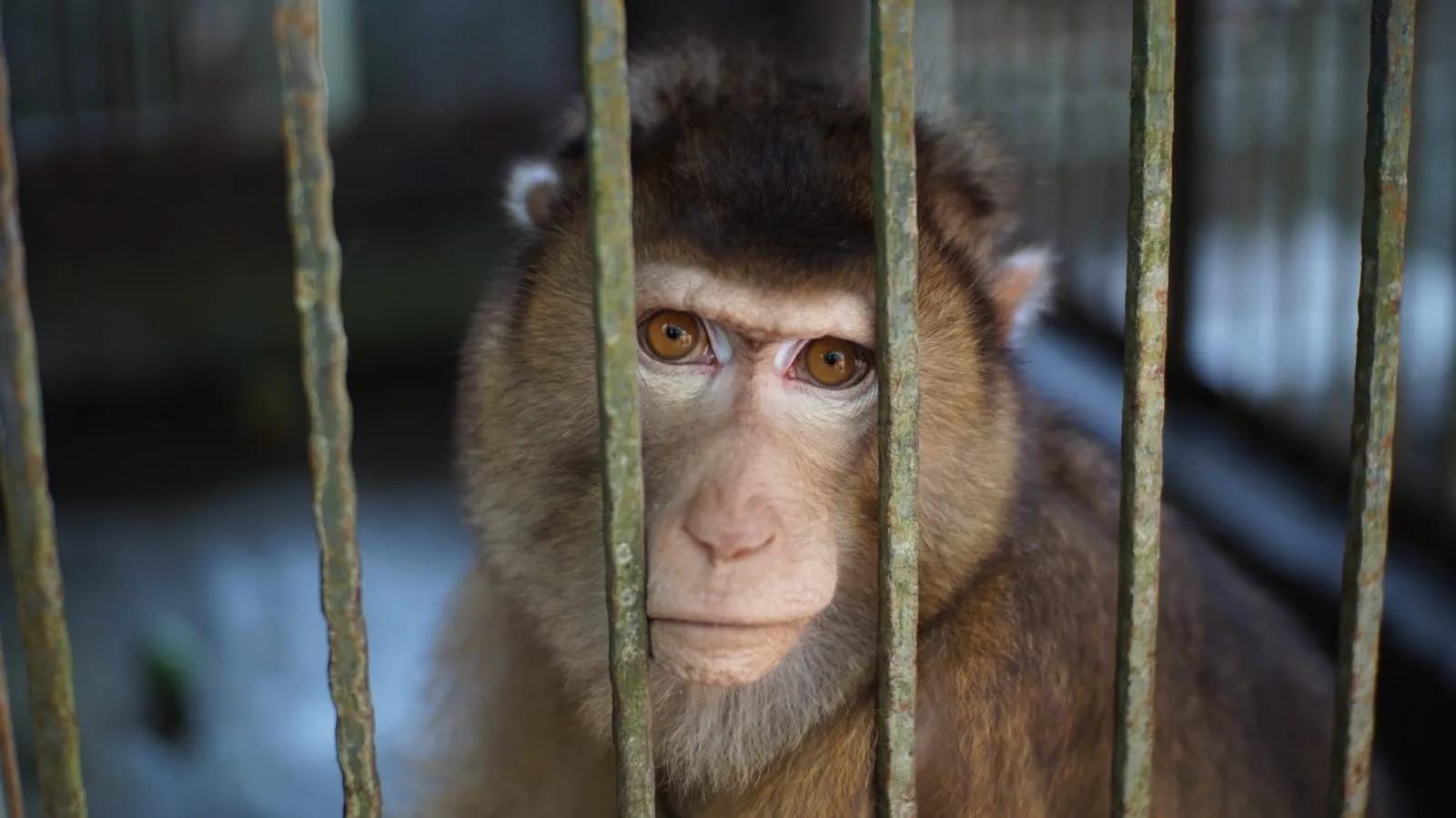
The Future of Parkinson's:
How to Reverse-Engineer the Brain
WrittenbyWendellOderkirk-Alvidrez | EditedbyMichaelGo | DesignedbyZacharyRentalaEnvisionaworldinwhichthedebilitatingsymptoms ofneurologicaldisorderssuchasParkinson’sdisease canbebroughtundercontrolwiththemereflickofa switch Thankstorecentbreakthroughsinthe researchofDeepBrainStimulation(DBS),thismay soonbecomeareality Thisarticleseekstoexplorethe innovativeclinicalapplicationsofDBSandthe remarkablepromiseithasintreatingawiderangeof disorders.
DBS,alsoknownasabrainpacemaker,involves placingmultipleelectrodeswithinthebrain. Followingthis,aneurostimulatorattachedtothe electrodescarefullypulsescurrentthroughoutthe system Similartoaheartpacemaker,theseelectrodes, andtheirassociatedneurostimulatorsinterruptthe irregularsignalsthatcausetremorsandother symptoms[1]
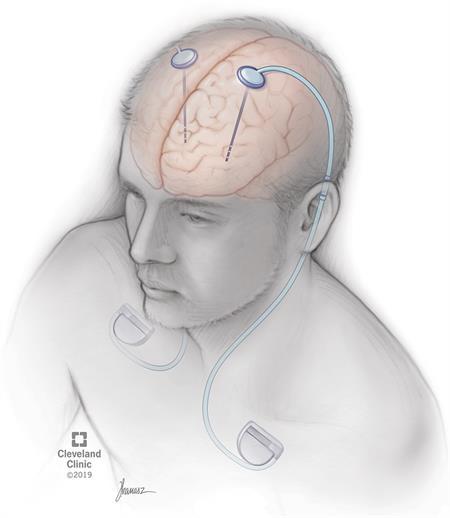
ThehistoryofDBSissomewhatfraught,filledwith questionsofunethicaltreatmentandadhocscience thataimedtoshockpeopleintofeelingbetter
AccordingtoHarizet al(2010),thehistoryofDBS startsmuchearlierthanpreviouslythought,with primaryresearchintoelectricalstimulationasa therapeuticstartingatTempleUniversityin1947.In the50s,thistherapeuticwasmostlyaimedat“curing” psychiatricpatients.Certainpsychologistsand neurosurgeonsatthetimecriticizedDBSforbeing associatedwith“theexcessesofpsychosurgery,”a referencetothehistoricalabuseofsurgical interventionsintreatingmentalillnessthatresulted innegativeconsequencesforpatients However,the technologyeventuallymaturedasitssafetyand effectivenesswereestablishedinvariousclinicaland researchstudies By2007,DBSwassuccessfullybeing usedtoimproveconsciencelevelsinapatient, improvetremorsassociatedwithmultiplesclerosis andParkinson's,andincreasedthesurvival probabilityofpatientsto51%over10years
ThescienceofDBSiscomplex,requiringtheuseof engineering,cutting-edgebrainimaging,andan intimateunderstandingofthehumanbrain To understandhowDBSmanagestoaffectthese signals,onemustunderstandhowneuronsinthe brainsendsignalstootherneurons Therearetwo waysneuronscancommunicatewitheachother: viatheuseofchemicalneurotransmittersor directlyallowingchargedionstoflowbetween neurons.Inbothcases,thesignaltravelsacrossa shortdistance,calledthesynapse.DBSfocuseson electricalsynapses,whicharemorecloselyrelated tomovement.DBSworkstoinhibitelectrical signalsinagivenareaofthebrain,especially areasknowntoshowincreasedactivityduring tremors[2] Duringsurgery,alead-containing electrodeisledintothebrainandthearea needingtreatment Certaindiseasesrequire differentareastobestimulated
Forexample,stimulationintheventralcapsuleor ventralstriatumhasbeenusedtotreatobsessivecompulsivedisorderandtreatment-resistant depression[3] Alzheimer’sdiseasenecessitates treatmentinthenucleusbasalisofMeynert,an areaofthebrainwhosedeteriorationisassociated withtheprogressionofthisdisease DBSdoesthis bystabilizingtheelectricalsignalssentoutbythe neurons,allowingdiseasedcellstohavehigher signalfidelity[4] Ingeneral,areasthatareknown tobehypoactiveorhyperactivearethosethatare giventhemostfocus.Specialreal-timeMRI technologyhelpssurgeonsintheplacementofthe lead.TheexactphysiologyofDBSispoorly understood,andthelocationsoftreatmentwere foundthroughtrialanderror.However,itis knownthatvoltageandpulsedurationaffect whichneuralelementsareactivated:lowervoltageandlongerpulsesignalsleadtotarget neuralelementsbeingactivatedwithoutaffecting non-targetcellsthatarenotrelevantto treatment
WenowknowthatDBSmaybecapableofrelieving symptomsofmanydiseases,includingParkinson's disease,essentialtremor,dystonia,OCD,and depression Thereal-worldeffectsandoutcomesof thistreatmenthavemostlybeenpositive AccordingtoDallapizaaet al(2018),patients treatedwithDBSreporteda30-60%improvement inmotorscoreevaluations 60%ofOCDpatientsat Mt SinaiHospitalsawrelief[5] 50-70%ofdystonia patientsatUCLAHealthsawimprovement[6] However,giventhecomplexityofthebrain, unintendedsideeffectsarealwayspossible.Some patientsarenotsuitablecandidatesforthis treatment:thosewithbalancedifficulties,those withdiseasesthathavenotrespondedtoanyother treatmentinanyway,andthoseatriskforsurgery. Forexample,probesthatareplacednearthe thalamuscanleadtotheworseningofdepression symptoms,asthethalamusisessentialinnormal affectivefunction[7]
ThefutureofDBSismostlyrelatedtotheneedfor longerbatterylifefortheneurostimulator,better placement,andwirelessandadaptive rostimulators,allowingfortheconservationof gyandresourceswhileimprovingpatient fort Currenttechnologiesarelimitedto rostimulatorsthatarepermanentlyactivated. ptiveneurostimulatorscanadjustthetiming patternofelectricalsignalsfromthebrainor oussystem.Thesesignalsarebeneficial usetheychangebeforesymptomsemerge, wingformoreproactivetherapy.Thismeans theDBSstimulusdoesnothavetobemanually ngedandupdatedcontinuouslywithmultiple ointments Withitsdirecttreatmentfocusedon sicallychangingtheelectricalsignalsinthe brain,DBSrepresentsatrulygroundbreaking advanceinneurotechnologyandtransformingthe livesofcountlesspatients
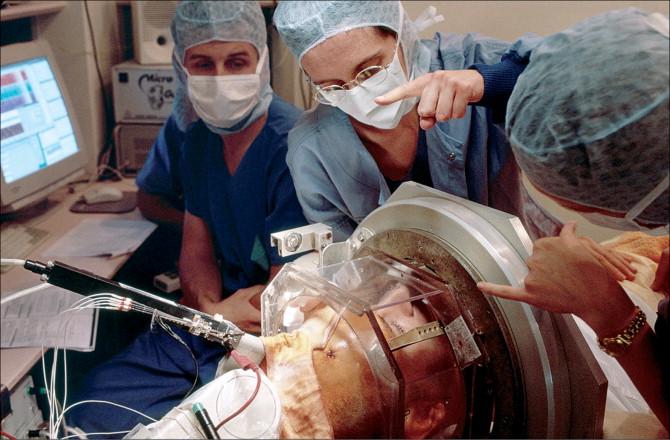
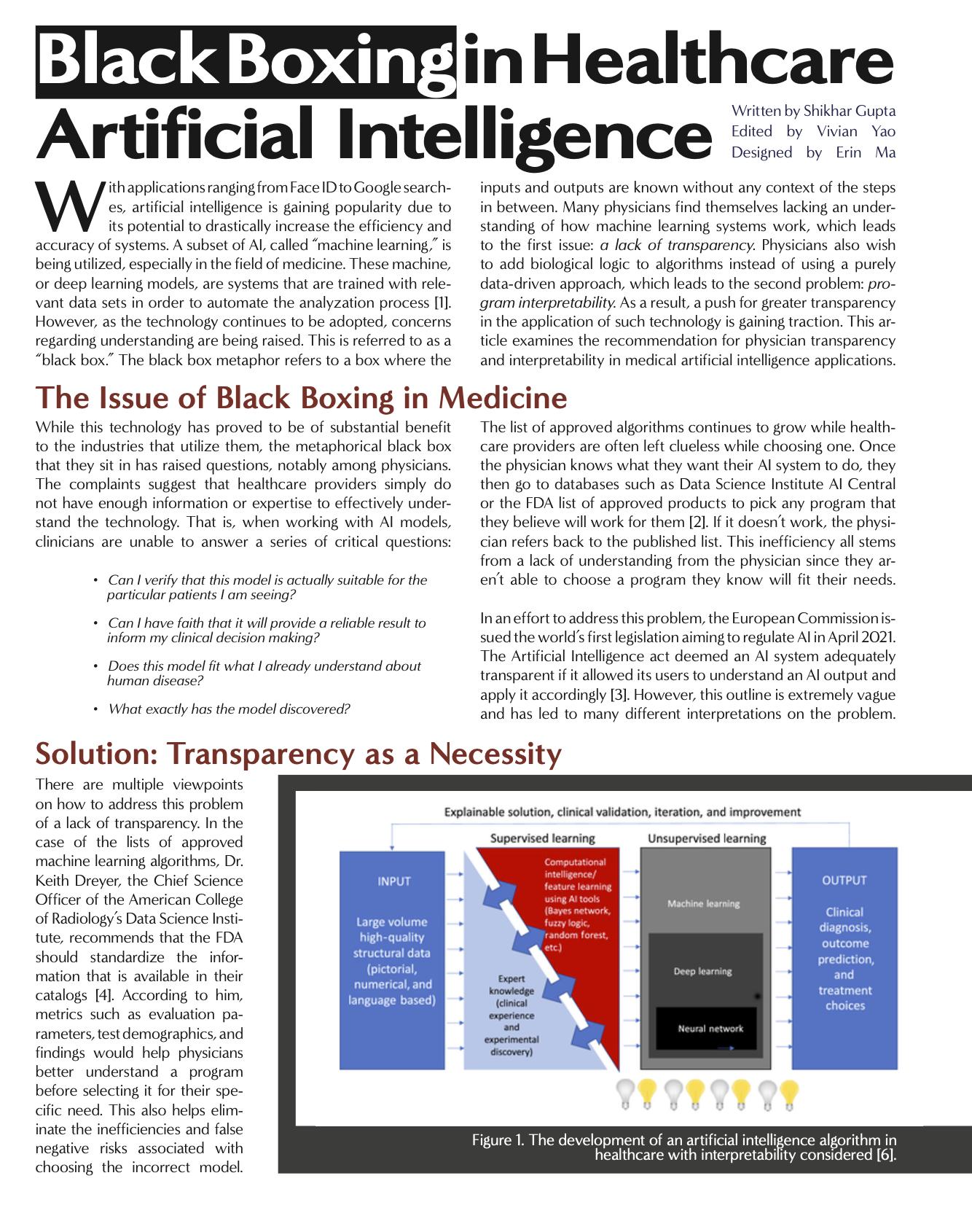
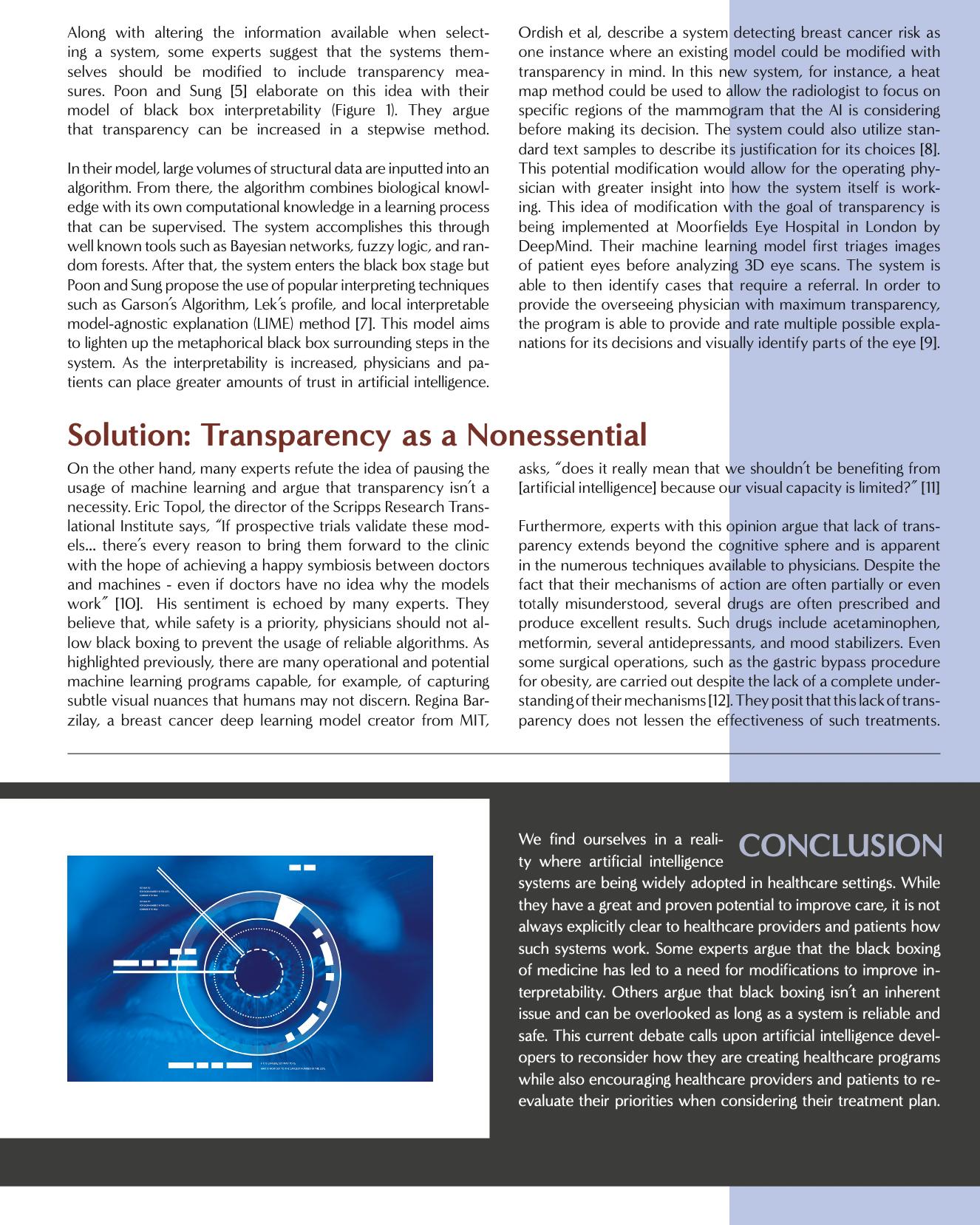
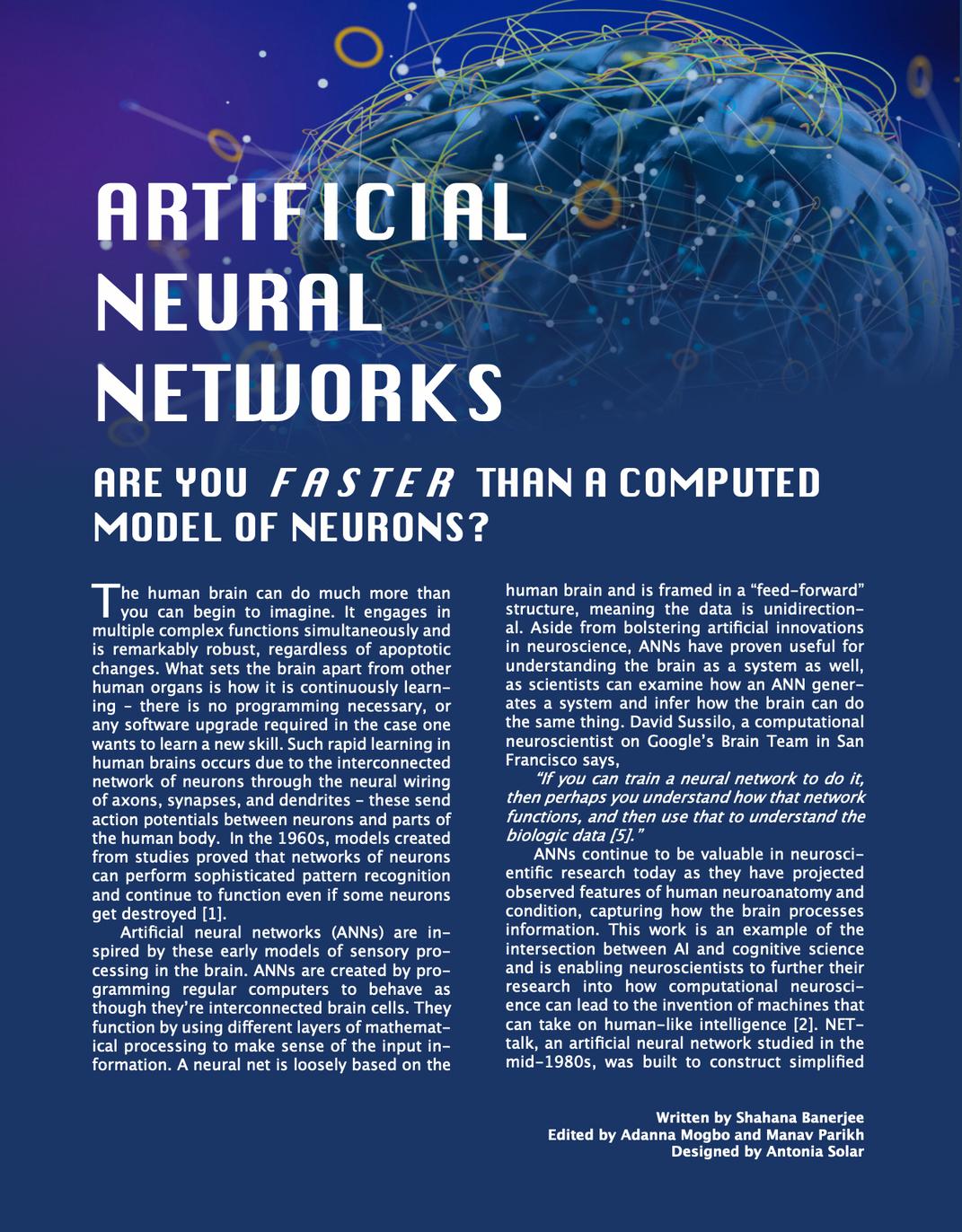
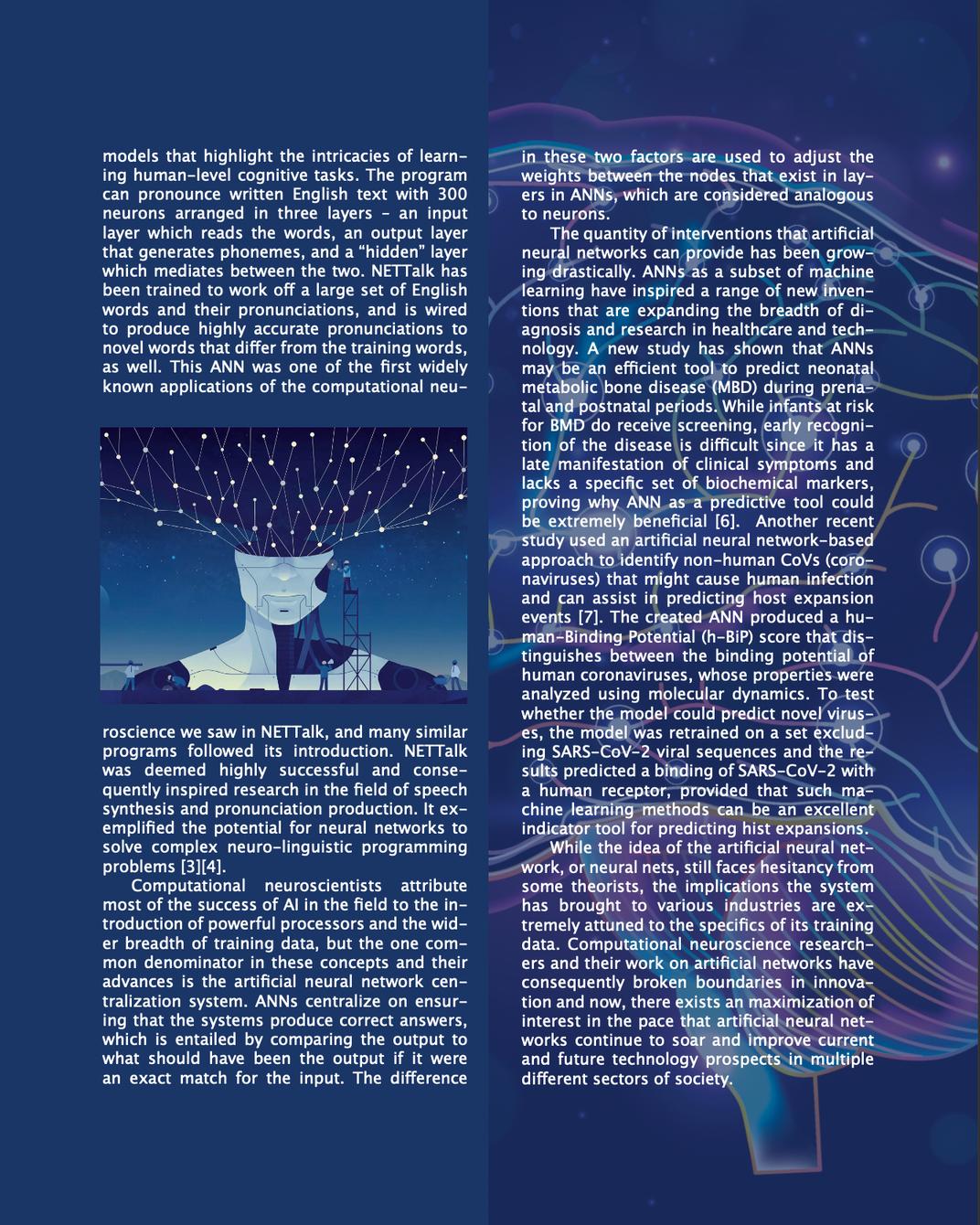
Leveraging AI in Radiomics
Written by Kayla Patel Edited by Shikhar Gupta, Shahana Banerjee, and Anagha Gouru Designed by Erin MaHealthcare is currently being revolutionized by technology. Digital solutions are being applied to every aspect of medicine. Artificial intelligence and radiomics specifically are being used to diagnose diseases and conditions affecting millions of people worldwide. How do AI and machine learning work to analyze scans? Is it truly accurate? And will this replace human image analysis altogether?
An Introduction to AI and Radiomics
Artificial intelligence is taking the world by storm, with the rise of self-driving cars, Amazon’s Alexa assistant, and ChatGPT. However, when looking at how AI can be applied to diagnostics, we must go back to its fundamental definition. Artificial intelligence definitively is a field that combines computer science with large sets of data to solve problems; machine learning, a subset of AI, specifically uses data and algorithms to imitate how humans learn with improving accuracy [1]. If we view the difficulty of image analysis as a problem that needs to be tackled, machine learning emerges as a perfect solution. Training algorithms to look at images and diagnose conditions from appendicitis to breast cancer by teaching them with millions of existing MRIs, CTs, PET scans, and ultrasounds can work through the more powerful image analysis of radiomics.
Radiomics is the more unfamiliar concept but is already applied to medicine and studies being done at several institutions, including the University of Pennsylvania. It is specifically applied to radiology, aiming to “extract a large number of quantitative features from medical images using data characterization algorithms” [2]. The results have the potential to identify aspects of diseases hard to diagnose by simply looking at the scans, ultimately aiding in the diagnosis process. It operates via the steps listed on the right [3]:
Radiomics has already seen great success, especially in oncology due to the abundance of images for data and large amounts
1. Image processing: images are analyzed using algorithms such as contrast and edge enhancement [4] to make the images as usable as possible. This allows for better anaysis later as it is easier to identify specific characteristics.
2. Image segmentation: areas or volumes of interest (genrally referred to as “regions of interest”, or ROI) are idetified [5]. Machine learning can be used to automate certain parts of this process, although some operator in put may be required for more complicated diseases like cancer [6]. For example, if looking at images of cardiac tissue, ROIs may include specific areas of the heart.
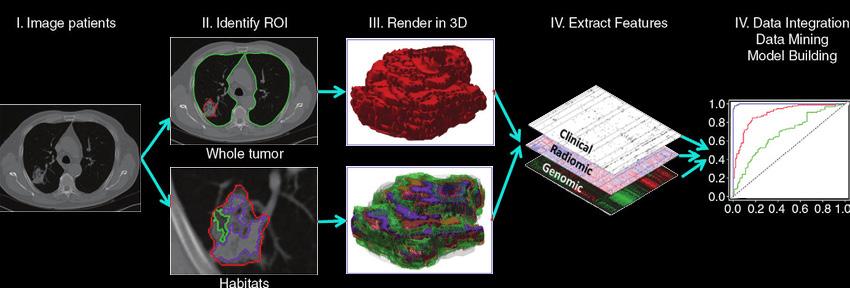
3. Features extraction and qualification: specific features of what is in the image are determined and analyzed volume, shape, surface, density, location, and relations with the surrounding tissues [7].
4. Model construction and classification: once radiomicfetures of interest are chosen, they are used to predict target variables in the present like presence of adisease or type of tumor - or in the future, such as response to treatment or the time for recurrence [8]. Machine learning can again be applied here to learn and train on the relationship between radiomic feature inputs and target variables based on examples.
Figure 1: Flowchart shows the process of radiomics and the use of radiomics in decision support. Patient work-up requires information from disparate sources to be combined into a coherent model to describe where the lesion is, what it is, and what it is doing. Radiomics begins with acquisition of high-quality images. From these images, a region of interest (ROI) that contains either the whole tumor or subregions (ie, habitats) within the tumor can be identified. These are segmented with operator edits and are eventually rendered in three dimensions (3D). Quantitative features are extracted from these rendered volumes to generate a report, which is placed in a database along with other data, such as clinical and genomic data. These data are then mined to develop diagnostic, predictive, or prognostic models for outcomes of interest.
The Existing Issues with Radiomics
The actual process of using radiomics presents some challenges. First of all, there is a need for extremely high-quality images for the analysis to actually be performed; should this not be the case, or if correction protocols cannot be applied, then radiomics isn’t particularly helpful. Additionally, with image segmentation, a necessary step to determine ROI on the images, it is difficult to actually perform this segmentation on larger datasets should AI not be used. This automation is needed because of the time-consuming, potentially inaccurate nature of manual segmentation that can’t necessarily be replicated in the way that AI can.
A major issue that radiomics faces is class imbalances, particularly for studies looking at rare conditions in which it “is seldom that the condition of interest has the same prevalence within a cohort as does lack of this condition.” In simpler terms, classifiers that are not necessarily accurate are applied to more niche conditions, sometimes making complex conditions more simplified for the sake of classification. However, this may be clinically useless, even if the subsequent analysis is accurate in some
Is it worth it?
If such image-analysis models have a great potential for inaccuracies when provided with too many input parameters or data points, do the consequences to patients and healthcare outcomes override any benefits? Should a model classify images incorrectly, what are the ramifications of unidentified tumors, or misdiagnosed and undiagnosed diseases? Obviously, these are not preferable outcomes. However, it could take months and potentially years to train a specific model and, while there can be some similar aspects between models for different conditions, in order to ensure maximum accuracy, there must be proper training and validation datasets.
Additionally there is the increasingly realistic idea of AI replacing the doctors currently handling image analysis. Although image analysis is not fully manual, there are those that worry about the role of AI in fields like radiology, where algorithms and models can be trained to do the job, but potentially better and faster. The use of machine learning can potentially reduce the error rates within radiology that are as high as 30%. Sometimes the algorithms can even outperform humans: for example, Google created a platform that outperformed a group of radiologists when determining the presence of lung cancer, diagnosing patients with
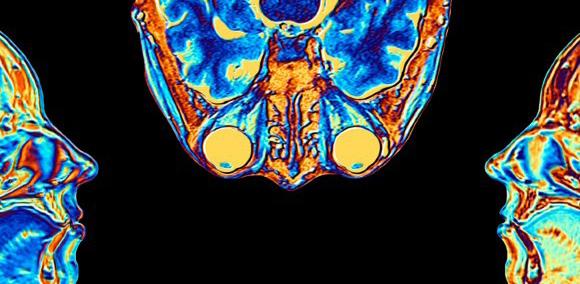
Conclusion
AI is the future of healthcare, and creating combined solutions is how we move medicine forward. The medical problems affecting people have not changed, but how we approach those problems has. Healthcare providers are no longer confined to the limits of their human abilities, but can now provide care augmented by digital solutions. Computational medical solutions like AI and radiomics have the potential to provide more accurate care on a much larger scale, reaching increased numbers of patients and truly helping as many people as possible.
regards. There can also be problems with the models provided by machine learning. Models can both overfit or underfit their approximations. Overfitting is when models can’t generalize information due to large amounts of input radiomics parameters or excess degrees of freedom, resulting in extraneous information being presented in the model, such as random fluctuations or image noise. These overfit models give correct results for the training set of images through which the model learns how to classify images, but for points outside the training dataset, the model is incorrect. There can also be underfitting in which a model cannot classify data correctly and is overly simplistic.
a greater level of accuracy and less false positives. The use of machine learning and radiomics could allow for more patients to be seen since models can process hundreds of thousands of images on a greater scale than physicians could. The use of image analysis models can bring healthcare to areas with fewer doctors and fewer resources, making healthcare offerings more equitable overall.
However, there are still numerous problems with the development of models, especially with obtaining enough data to actually create them successfully to the point that classifications and diagnoses are accurate. As such, it still seems that full replacement of physicians isn’t happening for quite some time. Furthermore, the implementation of AI and radiomics in healthcare doesn’t have to operate in extremes, with only humans or only technology analyzing scans. There can and should be a point of harmony in which the work of radiologists is made even better by the use of machine learning models to improve outcomes. At Beth Israel Deaconess Medical Center, the combined use of an AI model with trained pathologists led to correct identification of cancer in pathology slides 99.5% of the time, compared to 92% using only the AI model and 96% by pathologists alone.
Although AI is seemingly difficult to understand, it simply is a tool that takes medical images and their associated data, identifies areas of interest, and utilizes powerful algorithms to yield desired results. Through radiomics, AI can potentially have limitless possibilities when applied to medicine. However, issues with AI model accuracy and a lack of image data, among other problems, must be addressed in order for these technologies to be harnessed to their full potential. Should we reach that point one day, medicine will forever be changed.
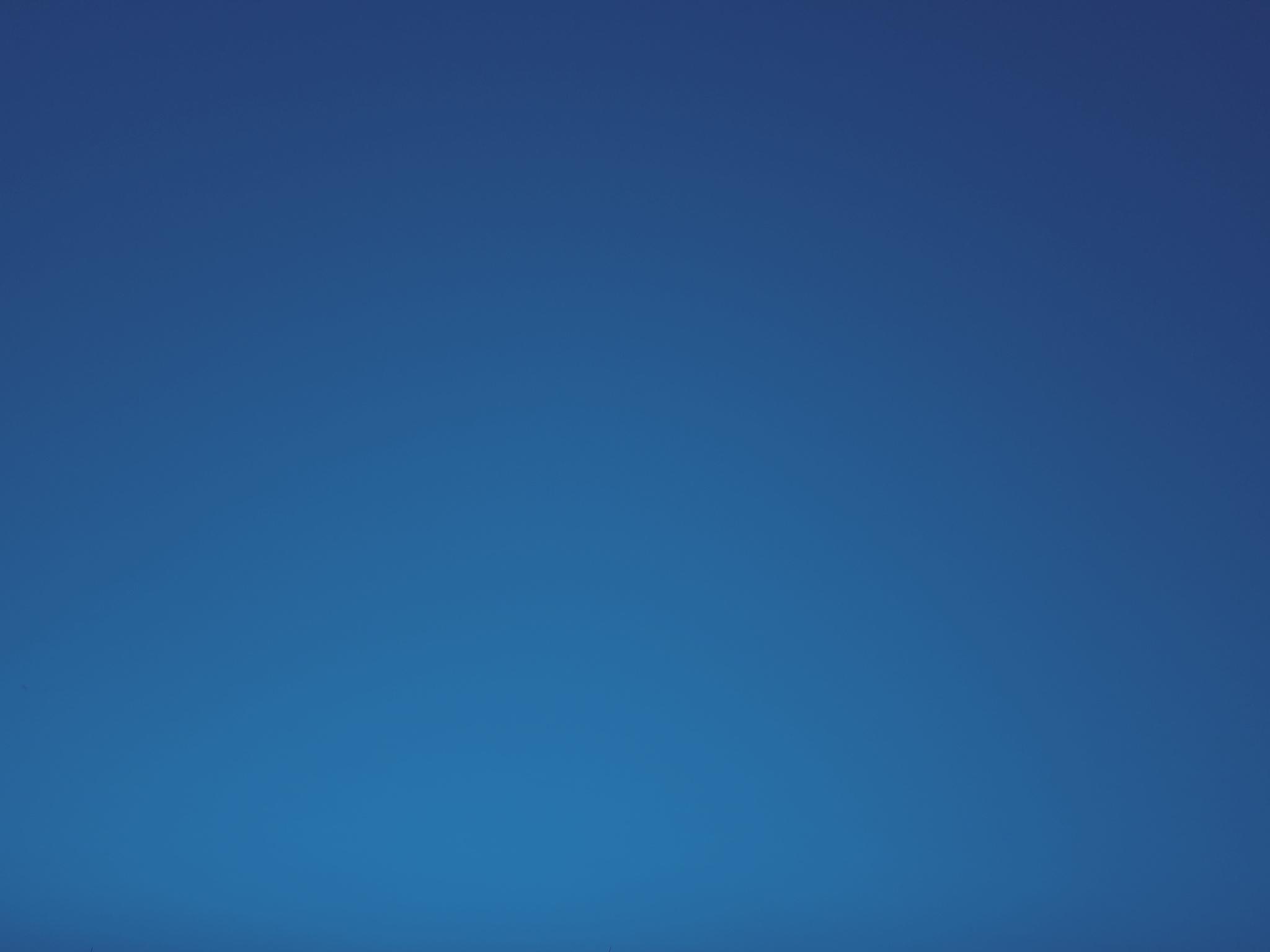
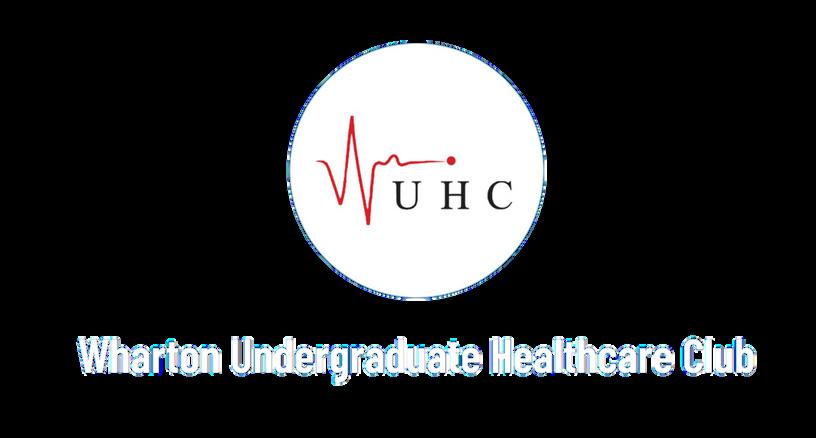