5.0 | Microscopy image of the bacteria
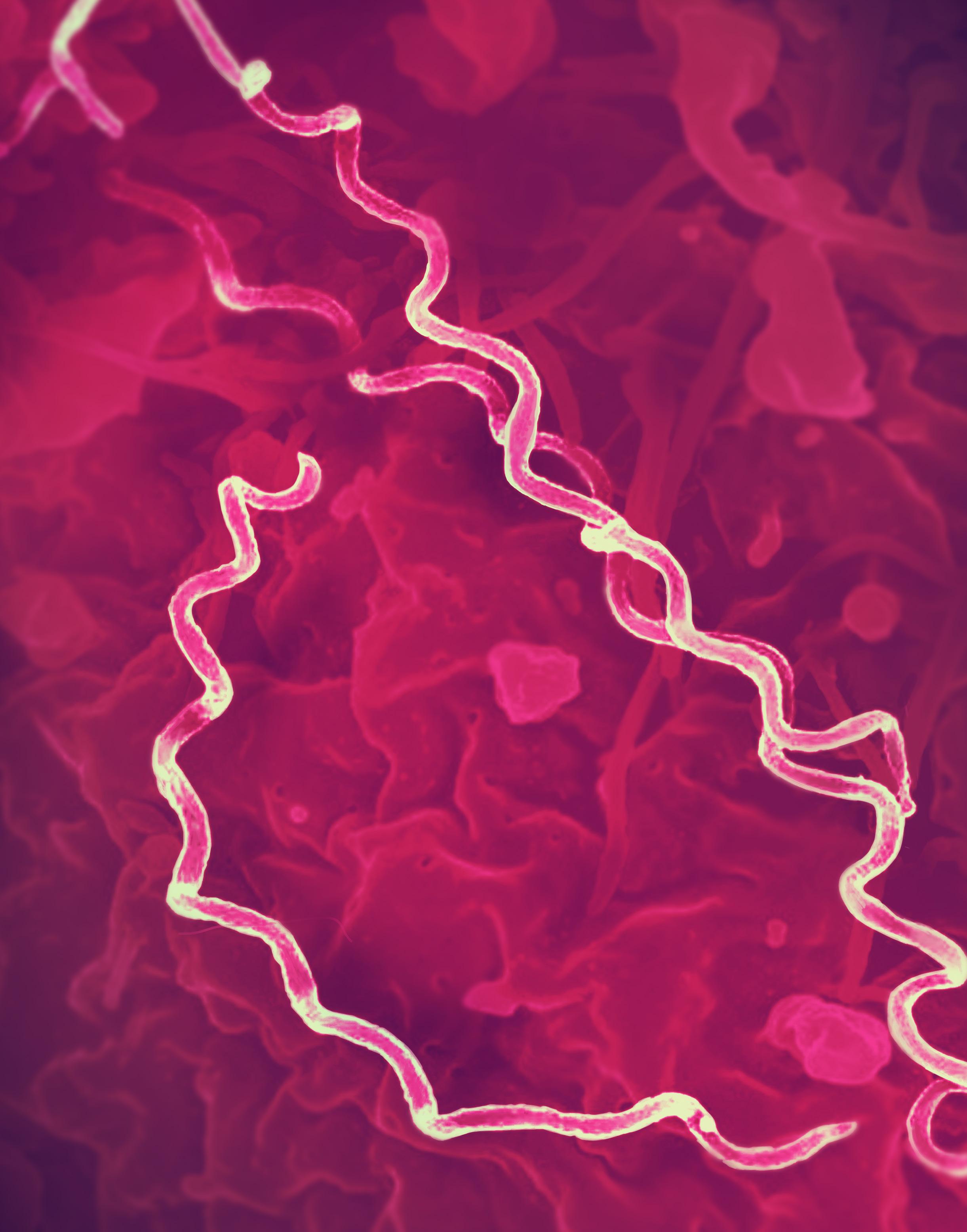
CHAPTER 5
5.0 | Microscopy image of the bacteria
CHAPTER 5
Here are a few essential terms commonly used in the biology of infectious agents. By the end of this chapter, you should be able to apply these expressions and know how they relate to other critical concepts.
Bacteria
Fungi
Helminths
Immunocompromised
Incubation Period
Koch’s Postulates
Microbiology
Non-Microbial Infectious Agents
Parasites
Prions
Protozoa
Viruses
Infectious diseases are caused by a diverse group of biological agents. While the types of infectious agents may vary, all pathogens are made up of biological molecules and can spread from one organism to another. Every infectious agent can cause either mild or highly severe diseases, and in some cases, no disease. It can be challenging to prove that a pathogen is the cause of a disease; Koch’s postulates were the first known attempt to describe the minimum evidence needed to establish this link.
Viruses are microbes that can only reproduce inside the cells of a host organism. Because they require their host cell’s machinery to reproduce, many do not consider viruses as “living.” Regardless, they are some of the most devastating pathogens and can spread quickly around the globe. Viruses are responsible for many common infections and devastating outbreaks, including Ebola, SARS-CoV-2, influenza, and measles.
Bacteria are single-celled prokaryotic microorganisms populating many habitats in our world and bodies. Bacteria are the most wellstudied type of microbe, and our understanding of their physiology allows us to identify them and to design therapies that specifically target them. Bacteria species vary in shape, but they all present a cell wall and asexual reproduction. Bacteria cause global scourges such as tuberculosis (Mycobacterium tuberculosis) and cholera
(Vibrio cholerae), as well as other ailments like diarrhea (Escherichia coli) and urinary tract infections.
Protozoa are a diverse group of singlecelled eukaryotes with complex life cycles, including several widespread parasitic organisms. As eukaryotes, protozoa can be hard to treat due to their cells’ similarities to human cells. These similarities, coupled with their complicated life cycles, make protozoa extremely resilient pathogens. The most wellknown and extensively studied protozoan family is Plasmodium, the parasite family that causes Malaria. Other protozoan families include Amoeba, Trichomonas, and Trypanasoma, which can cause diarrhea, vaginal infection and sleeping sickness, respectively.
Fungi range from single-celled to highly complex multicellular eukaryotic organisms which can be particularly dangerous to immunocompromised individuals. Pathogenic fungi’s eukaryotic nature and unique reproduction pathways make it very difficult to develop
therapeutics against them. Fortunately, most people exposed to these fungal species never get sick from them; most cases occur as opportunistic pathogens take advantage of individuals with weakened immune systems. Fungal families include Candida and Cryptococcus, which can cause candidiasis and cryptococcal meningitis.
Other infectious agents that cause severe human disease are either too large to be considered microbes or are not even organisms, such as helminths and prions. Helminths are worms that can significantly harm humans’ health after they enter the body, causing diseases such as Elephantiasis. Prions are misfolded proteins that can induce the abnormal folding of other proteins, having the potential to cause disease in humans and to spread. Unlike other infectious agents, they are just biological molecules and thus truly nonliving. Bovine Spongiform Encephalopathy (BSE), nicknamed “Mad Cow Disease,” is a devastating neurological disease in cows caused by prions that can spread to humans causing what is known as Creutzfeldt-Jakob disease (CJD).
Watch “Vick’s Video Corner ” as an entry point for this chapter.
By the end of this chapter, you will know the key features of infectious agents including their basic biology, how they reproduce, and the current strategies we use to tackle them. You will also get a chance to dive deeper into specific examples of pathogens in each group of infectious agents and the diseases they can cause.
It’s 1898, and you’re a high-ranking surgeon working in the US Army during the SpanishAmerican War. It’s a harrowing job, and although you don’t work directly on the battlefield, you’re still deeply aware of the dangers your colleagues face. In your role as a medical investigator working to save these men, it becomes increasingly clear that one of their most dire threats is not the battlefield – it’s disease. You decide it’s time to intervene.
Yellow fever is the leading cause of death during the war. This disease is characterized by vomiting, fever, headaches, and severe liver disease, as well as jaundice, which causes the skin to turn yellow. One-fifth of those infected with yellow fever suffer organ failure, often leading to death. Yellow fever has been documented in the US, killing up to 20 percent of a community’s population in especially deadly outbreaks and wreaking havoc on individuals who have little to no idea of how to control or stop its spread. You are well positioned to investigate as yellow fever has been the focus of some of your earlier work in the US. You create a task force composed of yourself and three other doctors – Aristides
Agramonte, James Carroll, and Jesse Lazear –and travel to Cuba to study the disease and to better understand how exactly it spreads.
Your team sets up a series of carefully designed experiments to test different modes of transmission, and narrow down the true source of the disease. Perhaps due to the seriousness of the situation, or maybe even the enthusiasm of your group, you all seem willing to take major risks in pursuit of your scientific and public health goals. You begin with experiments to prove that yellow fever is not passed through poor hygiene or sanitation practices. To do this, you round up a group of volunteer soldiers and, with the promise of a hefty sum of money, make the enormous request that they use soiled clothes and blankets, as well as handle
“… animalcules were in such enormous numbers that all the water… seemed to be alive.”
—Anton van Leeuwenhoek (1683), Dutch microscopist
other items of individuals infected with yellow fever. Aside from the discomfort of using bloodied blankets, these volunteers do not fall ill, and your team concludes that contact with contaminated items does not cause infection.
The task force moves on to test your most promising hypothesis, proposed by Cuban physician Carlos Finlay, which posits that the pathogen causing yellow fever is carried from person to person via mosquitoes. Lazear has worked with Finlay in the past, and your group becomes heavily invested in proving this idea once and for all. So, you carry out another very risky experiment, making mosquitoes feed on yellow fever patients and having them bite volunteers to see if these individuals become infected with the deadly disease. Among the volunteers is task force member Carroll, and you later learn that Lazear has also experimented on himself. Both men develop severe cases of yellow fever, and although Carroll survives, albeit with severe heart problems, Lazear tragically dies less than two weeks after he is bitten.
While you grieve for your friend, you also note that “his death was not in vain. His name will live in the history of those who have benefited humanity.”
You create a new experimental headquarters that you call Camp Lazear, repeating some of your previous experiments with even more carefully detailed controlled variables, experimental hypotheses, and exhaustive trials to ensure your data can’t be refuted. With the work and sacrifices of the many volunteers and task force members, you’ve been able to successfully prove that yellow fever is passed to susceptible individuals via mosquitoes. Additional army scientists quickly come up with ways to limit mosquito exposure and protect troops from the disease. Cases of yellow fever drop significantly
as a result of your work, and although you took risks that would be considered unethical in the modern age, you believe that your study participants and colleagues did not die for nothing. The advancements in our understanding of yellow fever yielded by their deaths has benefitted the whole world.
You are Walter Reed, a US army physician and the inspiration for the Walter Reed Army Medical Center and the Walter Reed Army Institute of Research, among many other locations and institutions. By following all lines of inquiry and rigorously following up on Carlos Finlay’s theory, you and your team have played an instrumental role in unraveling the mystery behind one of the deadliest diseases humanity has ever faced.
| Walter Reed and Juan Carlos Finlay y de Barrés. Photographs of the US Army physician Walter Reed (left) and the Cuban epidemiologist Juan Carlos Finlay y de Barrés (right). In 1901, Walter Reed led a team of epidemiologists to confirm Carlos Finlay’s theory that yellow fever is transmitted through a mosquito vector.
To effectively prepare for and respond to an infectious disease outbreak, scientists must understand the biology of the organisms that cause the outbreak. Most infectious diseases are caused by microbes, which are small pathogens that require a microscope to be observed. In order to successfully contain microbes, you first need to identify the pathogen that is causing the disease, then understand its characteristics and how it is able to spread. Similarly, to create a successful vaccine you need to know what parts of the pathogen, when isolated, would be able to stimulate the immune system without themselves causing illness. For many modern vaccines, you must understand the microbe’s core components and how they function. Thanks to centuries of work, we are more prepared to characterize and combat pathogenic threats than ever before. As we look to the future of medicine and outbreak science, a deep understanding of the biology behind infectious disease will continue to play a fundamental role in our prevention and response efforts.
The story of Walter Reed and the yellow fever research highlighted how important it is to understand the cause of disease. The knowledge and technology we have today have allowed us to reduce the challenge of identifying a diseasecausing microorganism or the way a pathogen was transmitted. However, even as early as the 11th century CE, distinguished physician Ibn Sina speculated that some small unit – what we now refer to as a pathogen – could be
passed between people and cause disease. In line with these beliefs, Ibn Sina prescribed 40day quarantines, which involve monitoring and restricting the movement of individuals suspected to have been exposed to contagious diseases in order to prevent their spread.
In the 1500s, the Italian researcher Girolamo Fracastoro introduced the formal concept of germ theory, which stated that very small organisms were the cause of disease and could be transmitted between individuals. Within a century of Fracastoro’s findings, other scientists were independently discovering key concepts about these mysterious diseasecausing agents.
German Jesuit priest and polymath Athanasius Kircher wrote the first known reports of tiny living organisms to be seen using a microscope. In one of his writings from 1646, he noted “an innumerable multitude of worms” in samples of vinegar and milk and eventually suspected that these small creatures may cause disease, writing his speculation that “a number of things might be discovered in the blood of fever patients.” He proved his theory in 1656 after finding microorganisms in the blood of bubonic plague victims, playing a key role in the origin of the germ theory of disease.
Despite Kircher’s prior work, Dutch microscopist Anton van Leeuwenhoek is often considered to be the first to describe microscopic living organisms and the “Father” of microbiologythe scientific study of microscopic organisms. Van Leeuwenhoek made his own microscopes, and in 1674, he began studying rainwater and reporting what he described as “animalcules,” meaning “little animals.” He observed so many that he noted “all the water… seemed to be alive.” Today, we classify these specific animalcules as bacteria, protozoa, and microscopic animals.
In 1762, this germ theory was expanded by Marcus von Plenciz, a Slovenian physician who hypothesized that each disease was caused by a different organism within the human body; this was only proven later as research in the field moved forward.
Cholera, an intestinal illness that wreaked havoc on humanity’s digestive tracts for hundreds of years, was initially believed to be transmitted through “bad air” from rotten organic matter. In 1849, John Snow, a British physician proposed that cholera was instead caused by a waterborne or foodborne pathogen, and in 1854, during a cholera outbreak in London, Snow collected enough evidence to confirm his theory. We learned about this in Chapter 2: Epidemiology.
In the 1860s, Louis Pasteur, French chemist and pioneer of modern microbiology and immunology, discovered that beverages like milk “spoiled” due to bacterial contamination. Pasteur also discovered that microbes would get deactivated or destroyed with a thermal treatment, which we now call pasteurization, which is the process by which food is treated with mild heat to kill microorganisms. These discoveries led Pasteur to think that microorganisms could be the cause of disease in humans, further supporting modern germ theory.
From 1879 to 1884, German scientists worked on more systematic methods for the identification of disease-causing agents. Building on the ideas proposed earlier by German physician Jacob Henle, Robert Koch developed the idea that microbes could be responsible for infectious diseases. Koch and Friedrich Loeffler established four criteria known as Koch’s postulates applied to understand the relationship between a microbe and a disease (Figure 5.2). Koch refined these and published them in 1890.
3
5.2 | Koch’s Postulates. Robert Koch laid out four criteria that a pathogen must meet in order to be considered the cause of a given disease.
Koch’s postulates state the following:
1. The microorganism must be only found in individuals with the disease and not in healthy individuals.
2. The microorganism isolated from the individuals with the disease must be cultured (grown in the lab under sterile and controlled conditions).
3. It must be possible for a healthy individual inoculated with the cultured microorganism to fall ill with the disease.
4. It must be possible to isolate the microorganism from a newlyinfected individual, and the isolated microorganism must match the original microorganism.
Koch applied these principles to discover many disease-causing pathogens including Bacillus anthracis, the cause of anthrax, and Mycobacterium tuberculosis, then known as “tubercle bacillus,” the cause of tuberculosis.
Koch’s framework marked the beginning of a movement to create widely-accepted definitions for diseases that could be referenced by scientists around the world. Criteria like Koch’s postulates were a gold standard for years, allowing scientists to root all of their microbial research in a shared understanding of basic biology. However, nowadays, and even back when Koch established his criteria, it sometimes became challenging to satisfy every single postulate. For example, Koch discovered asymptomatic carriers of some bacteria, suggesting that some infected individuals were not presenting the disease. We continue to see this phenomenon occurring for many
pathogens today. In 1905 Koch received the Nobel Prize for Physiology or Medicine for his many scientific contributions.
Every day, as we encounter living things in our ecosystems, like other humans, animals, and plants, we also come across a range of life forms that we can’t see with the naked eye. These mainly include microorganisms and other microscopic agents that come in various shapes, sizes, and classification categories. These are known as microbes.
FIGURE 5.3 | Infectious agents. We have classified all of the infectious agents into two main categories: microbial and non-microbial. Microbial agents include viruses, bacteria, protozoa, and fungi, while non-microbial agents include helminths and prions. Viruses and prions are uniquely acellular and non-living, while all other pathogens are living organisms made up of cells. Examples of each of the leading infectious agents that can cause human diseases include HIV, which causes AIDS, Mycobacterium leprae, which causes leprosy; malaria-causing plasmodium, tinea which causes athlete’s foot, organ-damaging tapeworms; and the prions that cause CreutzfeldtJakob disease (CJD). Note: The size of the infectious agents presented here is not to scale.
Most infectious agents are microbes. The tiniest known microbes are viruses; however, they require a host to reproduce and thus are not even universally considered living. Bacteria are single-celled prokaryotic organisms found in every habitat on earth, including our bodies. Protozoa are a vast array of singlecelled eukaryotes with complex life cycles. Fungi include single-celled and multicellular eukaryotes, which also take many forms and have intricate life cycles.
Microorganisms are the most diverse group of organisms; altogether, they comprise more than 85% of the recorded biodiversity of all species. New estimates suggest there could be a trillion species of microorganisms on Earth. While microorganisms typically have a negative connotation, they are critical to maintaining human health and the functions of daily life. For example, bacteria in our gut produce necessary vitamins, and yeast is what makes bread rise. However, our attention in the field of outbreak science is often focused on the microorganisms that can be harmful to humans – the pathogens that can threaten our health.
There are other infectious agents that are not classified as microbes due to their differing size or molecular nature but can still cause severe diseases in humans. These non-microbial infectious agents include helminths, which are parasitic, non-microscopic worms, and prions, which are microscopic misfolded proteins that cause disease. Figure 5.3 shows this general classification system, as well as an example of a disease caused by each of these infectious agents.
When we include viruses, bacteria, fungi, protozoa, and helminths, there are about 1,400 known species of pathogens that can infect humans. Pathogens have shaped the course of human history and frequently cause serious global crises. Throughout history, the medical and scientific communities have relied on the biology of pathogens to design strategies that protect people from contracting illnesses and heal those already afflicted. In the next section, we’ll outline the biological features that make these different pathogens unique while highlighting a few common diseases they may cause.
1. What does microbiology study?
2. How did scientists first discover microscopic organisms, and how did they determine that these were the cause of some infectious diseases?
3. What are the four criteria of Koch’s postulates?
4. What are the different types of pathogens?
Measured to be about one-hundredth of the width of a human hair, viruses are the tiniest known microbes. They are found everywhere, with hundreds of virus species known to infect humans and an estimated 10 nonillion (1031) viral particles on earth – more than all of the planet’s cellular life forms combined. Viruses are ancient; the oldest identified human virus is 30,000 years old, and there is evidence of viruses integrating into mammalian genomes tracing back tens of millions of years.
As we have mentioned before, viral replication is dependent on the cellular machinery of a host cell. Unlike living organisms, they do not make energy or maintain homeostasis, which is the ability to balance physiological conditions necessary for survival. They may have even preceded the formation of cells, according to various hypotheses that viruses could be the ancestors of some organisms living on Earth today, implying that they may be “living” in some ways.
The vast majority of viruses are nonpathogenic to humans, meaning that they do not cause disease in humans. However, the subset of viruses that infect humans can cause serious diseases and huge outbreaks. Viruses have caused many diseases that you may know of, from COVID-19, to the common cold, to Ebola. Many viral outbreaks have been among the biggest threats to public health because of their quick replication times and our relative lack of effective viral treatments. Therefore, it is crucial that we fully comprehend how viruses operate when thinking about outbreak science as a whole. Understanding the biology of viruses – through a field of study known as virology – can help us mitigate, and hopefully
even prevent, future outbreaks. This section will discuss virus structure and biological characteristics and explain how they infect host cells to reproduce. We will also introduce how we can use our knowledge of the common features of viruses to mitigate viral outbreaks.
As introduced in Chapter 4: Fundamentals of Genetics, viruses vary in complexity and size, but every virus follows a basic 3D structure blueprint. A viral particle is known as a virion, and it is composed of genetic material encased in a protein shell called a capsid. Most, but not all, virions have an outermost layer called an envelope, which is studded with various envelope proteins. These proteins come in direct contact with the outside world, and they help the virus bind to receptors on the surface of host cells so that they can enter the cell.
Compared to other microorganisms, viruses have exceptionally small genomes, typically between one to tens of thousands of nucleotides long, but some can be a few hundred thousand nucleotides long. Viral genomes can be made of either RNA or DNA, and they can be either single- or doublestranded. As their genome size indicates, viral genomes typically consist of fewer than 100 genes, which is only a handful compared to bacterial genomes, which range from 600 to 10,000 genes, or the human genome containing around 20,000 genes. Despite their limited space, viral genomes carry a lot of information and encode for critical proteins that maintain their life cycle. As viruses invade host cells, they use the translation machinery of the host cell to make the proteins needed for viral replication.
There are two types of viral reproduction cycles seen in viruses. The most common cycle is the lytic cycle, during which the process of cellular hijacking and viral replication is accomplished through five steps.
1. Attachment: The lytic cycle begins when the virus attaches on to the host cell’s receptor.
2. Penetration: The viral genome then enters the cell.
3. Replication: The virus redirects the host cellular machinery to replicate the viral genetic material and produce viral proteins.
4. Assembly: The new ‘daughter’ virions assemble within the host cell.
5. Lysis: After making enough copies of itself, the virus lyses – or bursts open – and the cell releases a fresh batch of new viral copies.
The host cell dies after lysis, and its cellular remnants scatter, which is why viral infections can be so devastating to the host. Once the new viral copies are freed, they go on to infect new cells through the same cycle of attachment, penetration, replication, assembly, and lysis.
Some viruses follow the lysogenic cycle; instead of immediately reproducing and lysing the cell after attachment and penetration, the viral genome integrates into the host’s genome and remains latent or dormant for an indefinite period of time. After this integration step, each time the host cell matures and replicates over time, both the host genome and viral genome are replicated.
The viruses that undergo the lysogenic cycle usually remain dormant for long periods of time until the host cells are triggered by other
stressors and environmental pressures, such as depletion of nutrients, which then signal the virus to enter the lytic cycle and make new viral copies. The ability of a virus to remain inside a cell dormant without being active for long periods of time is known as viral latency. When a virus reactivates, it enters the lytic cycle again and replicates (Figure 5.4).
The herpes simplex virus, which causes herpes, and HIV, the retrovirus that causes AIDS, are two viruses that can integrate into the host’s genome, and they can remain dormant for years before replicating. When a retrovirus infects a host cell, the cell converts the viral RNA into DNA through reverse transcription, and the viral DNA integrates into the host’s cell DNA. The integration of the viral genomes into the host’s genomes makes it very challenging to treat. As you can imagine, the virus sticks around in a very efficient way by “hiding” within the host, hindering our ability to fully eliminate the infection.
The lytic
is generally described by 5 steps: (1)
(2) Penetration, (3) Replication, (4) Assembly, and (5) Lysis. In the lysogenic cycle, the viral genome is integrated into the host genome and replicated for some time prior to viral assembly and lysis. The lysogenic cycle’s dormant period can last for indeterminate lengths of time.
Viruses have many varied properties governing how they behave in a human host’s body, such as their incubation period or the number of days between when a microbe infects a host and when the host shows symptoms. The incubation period for viruses can range from just one or two days to several months. Diseases caused by viruses with short incubation times are of particular concern for both individuals and health systems during epidemics.
Although a swift incubation period can appear beneficial when the outcomes are positive, such as a quick recovery from a fever, the same turnover time can be dangerous when dealing with viruses that often have more deadly outcomes. For example, consider a person is infected with a viral hemorrhagic fever such as Ebola, which has an incubation time ranging from 2 to 21 days. In that case, their symptoms can escalate from relatively benign to fatal in a matter of days.
During a viral infection’s incubation period, the virus’s primary task is to replicate at very high rates within the infected individual. We often find a high concentration of a virus in places like a person’s mucous membranes, because these surfaces are frequently at the interface between the outside world and your body, like your eyes, nostrils, mouth – even genitals. This means that hosts are very likely to pass the infection to someone else when they expel mucosal fluids through coughing or sneezing. Further, the high concentration of the virus, coupled with its physically small size, can increase its airborne transmission. Although other types of pathogens can travel swiftly from person to person, the high replication rate of viruses coupled with their small sizes can explain why many highly transmissible infections we see today are caused by viruses that swiftly become widespread.
Since many viruses can replicate and be transmitted so quickly with devastating implications, it’s crucial that we utilize tailored strategies to combat the spread of viral disease as soon as we see signs of an outbreak. Thankfully, vaccines against viruses have been one of the most effective medical tools in human history.
Vaccines have played an enormous role in limiting the spread and severity of viral illnesses. As we will learn more about in Chapter 9: Vaccines and Immunizations, vaccines typically operate by safely exposing the immune system to a portion of a virus, either by direct injection of a deactivated (and therefore no longer dangerous) part of the virus into an individual, or by triggering the immune system to produce a non-infectious copy of the virus itself. This allows the potential host to develop an immune response without spreading the disease. Both methods allow the immune system to defend the body more effectively when re-exposed to the real virus, preventing severe, and possibly even fatal, symptoms. Vaccines have helped eradicate smallpox (reduce to zero cases in a global population) and have the potential to eradicate some of the worst viral diseases in history including measles and polio.
Viruses can also be treated after exposure and/ or infection through the use of antiviral agents. Because viruses use the same parts of the cell to replicate as the cells they are infecting, and some integrate in the host genome, it has been difficult to find and attack targets that are not also important to the host. However, there have been relatively recent breakthroughs in developing medicines that mimic our immune systems. We’ll learn more about these different approaches in Chapter 8: Therapeutics.
Many infectious diseases with which you may be familiar are caused by viruses. For example, the influenza virus affects millions of people each year through the seasonal flu and is responsible for the infamous 1918 pandemic. Many pediatric illnesses that have decreased in prevalence since the advent of vaccines, such as chickenpox, measles, and mumps, are also caused by viruses. Viruses have even been found to cause diseases that were once thought to be non-infectious, such as Human Papillomavirus (HPV), which can lead to cervical cancer.
As discussed in Chapter 1: Emerging Pathogens, both new and existing viruses have the potential to cause serious disease and major outbreaks. In 2009, the Swine Flu pandemic, caused by
5.1:
Virus
Ebola Virus (EBOV)
Human Immunodeficiency Virus (HIV)
Human Papillomavirus (HPV)
Influenza A Virus (FLU A)
Zika Virus (ZIKV)
Caused
the Influenza A virus H1N1, wreaked havoc and caused over 250,000 deaths worldwide. In 2014, the Ebola epidemic caused over 11,000 deaths, primarily in West Africa. The COVID-19 pandemic, caused by SARS-CoV-2, has caused over 6 million deaths around the world. In Table 5.1 we provide just a few examples of viruses that have caused outbreaks, epidemics, and pandemics in recent years. We’ll then dive into the biology of a virus with which you are already familiar: SARS-CoV-2.
Scan this QR code or click on this link to enter a Virus Explorer where you can probe the structures of various pathogenic viruses.
Ebola Virus Disease (EVD) (organ failure) RNA, 19kb
Acquired Immunodeficiency Syndrome (AIDS) (suppresses the immune system) RNA, retrovirus, 9.7kb
Genital warts and cervical cancer (affects the uterine cervix and genitals) DNA, 8kb
Type A Influenza (lungs infections) RNA, 13.5kb
Zika Virus Disease (affects the nervous system and fetal tissue when present) RNA, 11kb
Note: Microscopy images shown in this table are electron micrographs which can show objects magnified between 1 to 50 million times their actual size. Electron micrographs are black and white, but sometimes these images are digitally manipulated to show color as seen here. Keep in mind that viruses are too small to have a color. The HPV virus is a computer generated image of a virus-like particle.
SARS-CoV-2, which is the abbreviation for S evere A cute R espiratory S yndrome Coronav irus 2, causes a respiratory tract infection known as COVID-19. As you are likely already hyper-aware, symptoms of COVID-19 include fever, chill, cough, difficulty breathing, fatigue, headache, and loss of taste and smell. In the worst cases, however, it can cause severe acute respiratory distress and even death. It is a highly contagious virus with an incubation period ranging from 2 to 14 days.
SARS-CoV-2 is an RNA virus in the coronavirus family, a name derived from the Latin word for crown, corōna, after these viruses’ crownlike appearance. Unlike our human genome, which is made up of double-stranded DNA, the SARS-CoV-2 genome is made up of singlestranded RNA. Coronavirus genomes encode four main proteins. The spike protein (S) plays a role in attachment and mediating cell entry; forming the studs on the outer layer of the virus, they give coronaviruses their crown-like appearance. The S protein on the envelope of the virus targets the ACE2 receptor to enter the host cell. The envelope (E) protein is the building block for forming the envelope of the virus. The membrane (M) protein forms the viral membrane and is the most abundant structural protein. S, M, and E are all embedded in a lipid membrane and together form the viral envelope. The nucleocapsid (N) protein is contained within the viral envelope and encases the RNA genome like a shell (Figure 5.5).
SARS-CoV-2 follows the lytic cycle, infecting cells by attaching to a receptor on host cells and ultimately hijacking the host cell’s machinery to make new viral proteins. The newly-made proteins and RNA then assemble
FIGURE 5.5 | Atomic 3D model of the external structure of the SARS-CoV-2 virus which causes the coronavirus disease first identified in Wuhan, China in 2019. Coronavirus genomes encode four main proteins, called S, E, M and N for short. In this model you can see the spike protein in turquoise and orange, the membrane in cobalt, the M protein in green and the E protein in pink.
themselves into a new copy of the virus, which breaks out of the host cell through a process known as “budding.” The cycle continues as newly-assembled virions of SARS-CoV-2 travel to infect other cells.
The COVID-19 pandemic has driven rapid advances in developing viral countermeasures, with significant and rapid advances in vaccine development; many of you have experienced these advances firsthand, through events like the development, approval, and widespread administration of the first mRNA vaccines, discussed in Chapter 9: Vaccines and Immunizations. Fortunately, these countermeasures have greatly reduced the number of severe cases of COVID-19, but their success is currently being threatened by new viral mutations.
1. Why do we say that viruses are non-living (two reasons)?
2. What is the advantage for the virus to integrate into the host genome?
3. Why are integrated viruses more difficult to treat than lytic viruses?
4. What is the primary task of a virus during the incubation period?
Bacteria are ancient microorganisms. In comparison to modern humans, Homo sapiens, who have only existed for about 300,000 years, bacteria have existed for over 3.5 billion years. When we fight bacterial pathogens, we’re not just up against an isolated organism – we’re battling with billions of years of diversity and evolution.
Bacteria are ubiquitous and cover most surfaces on planet Earth, from the deepest part of the ocean to the highest mountain tops, including on doorknobs, mobile phones, pencils, and everything else you touch every day. You may be surprised to learn that bacteria cover your body, both inside and out; the human body contains about 40 trillion bacteria, even outnumbering the 30 trillion human cells that make up your body. Scientists estimate that there are roughly 1030 bacterial cells in the world, and about 30,000 different species have been identified.
Although most bacteria are non-pathogenic, a wide range of bacteria has inflicted tremendous harm throughout history. Indeed, before the advent of antibiotics, they caused some of the most devastating epidemics in history, including the bubonic plague, cholera, syphilis, and tuberculosis. As bacteria have been able to develop resistance to the therapies we have used to combat them, some of these older threats have emerged as dangers once again.
Let’s start by learning about bacteriology, the branch of biology studying bacteria. As we do this, we will also examine what all bacteria — both good and bad — have in common to better understand how they might cause disease and how we can stop them from causing harm.
FIGURE 5.6 | Bacterial cell structure. Bacteria are unicellular prokaryotic microorganisms that lack a nucleus, instead keeping their DNA in the nucleoid. They present a cell wall in addition to a plasma membrane and some have a flagellum as part of their structure.
As detailed in Chapter 4: Fundamentals of Genetics, bacteria are single-celled, prokaryotic organisms that do not have a nucleus to store their DNA; their genome is instead commonly stored in the nucleoid (Figure 5.6). Bacterial genomes are typically large circular chromosomes that range in size from 130-thousand (130 kb) to 14-million bases (14 Mb). Separate from their primary chromosome, bacteria also contain small circular fragments of double-stranded DNA called plasmids, which carry non-essential genetic material. These plasmids replicate independently from bacterial chromosomes and allow for the passage of useful genes to surrounding bacteria, such as those that cause antibiotic resistance and allow bacteria to produce different toxins.
Bacteria have a number of interesting cell structures. Some bacteria have what is known as flagella, a tail that can whip around and
provide motility – the ability to move – to the bacterial cell. Some have pili, which you can think of as little bacterial straws that allow for the passage of genetic material to other nearby bacteria that aren’t necessarily related. In other words, bacteria have ways of sharing their DNA with their friends.
All bacteria are encased in a multilayered cover called a cell wall . Bacteria rely on their cell walls to protect against threats and allow for the selective uptake of nutrients. Bacteria are classified based on the characteristics of their cell walls, which also gives us insight into what antibiotics may mitigate a given bacterial infection.
We classify bacteria through a classic diagnostic test, the Gram stain , which reveals features of their cell walls. In this staining method, a crystal violet dye is applied to bacteria and rinsed out with ethanol. After this process, the color the bacteria appear depends on their cell wall structure, and based on this result can be classified as gram-negative or gram-positive (Figure 5.7).
Gram-negative bacteria cell walls contain three layers: an outer membrane, an intervening cell wall, and an inner (cytoplasmic) membrane. The inner and outer membranes are semipermeable, meaning they only allow certain molecules to pass through. Within the outer membrane, you will find lipopolysaccharides (LPS), large molecules consisting of a lipid and a polysaccharide. The intervening cell wall provides most of the structure of the cell and consists of peptidoglycan – a complex
of sugar and protein molecules – which protects the inner membrane. This wall acts as an exoskeleton (meaning “outside” skeleton), allowing the bacteria to maintain its structure even in conditions where it might otherwise lyse. When Gram stain is applied on the gramnegative bacterial cells, the thin peptidoglycan layer cannot successfully retain the dye, and it is washed out, leaving the cells with a reddish hue.
Gram-positive bacteria only have two layers in their cell walls: the rigid, peptidoglycan cell wall, and the inner cytoplasmic membrane. Lacking an outer membrane, gram-positive bacteria compensate for the lack of stability with extra layers of peptidoglycan in their cell walls. This extra-thick peptidoglycan layer binds to the Gram stain dye much more strongly, giving the cells a dark violet color. We will revisit the Gram stain and its implications for diagnostics in Chapter 7: Diagnostic Tests.
Scan this QR code or click
FIGURE 5.7 | Gram staining. A) These diagrams show the cell walls of gram-negative and grampositive bacteria. Gram-negative cell walls have three layers, which appear pink when Gram-stained, whereas gram-positive cells have two-layered cell walls and turn violet when Gram stained. B) Gramnegative Bacteroides fragilis cultured and stained pink by Gram stain, unable to retain the dark violet color. C) Microscopy image features bacteria stained with the Gram stain. Gram-positive anthrax bacilli are stained purple by Gram stain in a sample of cerebrospinal fluid.
FIGURE 5.8 | Bacterial classification based on their shape. Bacteria are classified in four main groups on the basis of their shape: Cocci, Bacilli, Vibrio, and Spirilla. In Outbreak Science, bacteria can be identified based on their shape when observed under a microscope.
A small number of bacteria cannot be captured by a gram-stain test. These are described as acid-fast bacteria , meaning they are resistant to decolorization by acids during laboratory staining procedures. This is due to the high mycolic acid content of their cell walls, which forms a waxy coat. Mycobacterium, including tuberculosis and leprosy disease-causing bacteria, are the most prominent acid-fast bacteria. Other bacteria are partially acid-fast, including Nocardia, which is responsible for a bacterial disease affecting the lungs, brain, and skin.
Bacteria are also classified into three major groups based on their shape 1) Cocci: spherical-shaped; 2) Bacilli: rod-shaped; and 3) Spirilla: spiral or curved-shaped (Figure 5.8).
There are two main forms of reproduction in living organisms: sexual and asexual. In sexual reproduction, two parent organisms are involved, and their genetic information is “mixed” to create genetically unique offspring. Humans are an example of animals that reproduce sexually, with sex cells from two different parents fusing to produce offspring. In contrast, there is only one parent in asexual reproduction , who copies itself and gives rise to genetically identical offspring. Bacteria are an example of organisms that reproduce asexually with just a single parent. As we will see below, some organisms, such as Plasmodium, protozoa, can engage in both sexual and asexual reproduction.
FIGURE 5.9 | Binary fission. Most bacteria propagate through binary fission, the bacterial asexual cellular reproduction process. Through this process, the bacterial cells double their size, and then they split in two.
The life cycle of a bacterial cell begins with the parent cell doubling its cellular components and growing to twice its normal size. DNA replication is part of this cellular duplication process, beginning at a spot along the circular chromosome known as the “origin of replication” and continuing out in both directions around the circle. After everything has been replicated – including the bacterial DNA – the center of the swollen cell begins to cinch, eventually pinching into two cells, with one copy of the genome and plenty of cellular machinery in each. This method of reproduction is known as binary fission (Figure 5.9).
Bacteria often replicate much faster than our cells; for example, Escherichia coli replicates about every 20 minutes. Even though 500 million bacteria can fit into a grain of sand, uncontrolled bacterial replication would be prolific enough to cover the earth’s entire surface in only two days. Luckily, many components of their surrounding ecosystems keep the bacteria in check and under control.
With bacteria’s great biodiversity, they can have a vast array of different interactions with hosts, ranging from benign to deadly. Most of the bacteria in the human body are commensal, meaning they do not cause harm; some may even be mutualistic, meaning both the bacteria and the host benefit from their interaction. These beneficial bacteria form a part of the diverse community of microorganisms found in human bodies known as the microbiome. Your microbiome plays many important roles in your body’s natural functions, such as helping break down the food we eat, and even preventing colonization by pathogenic bacteria, fungi, or viruses. The bacteria of the human microbiome can directly kill pathogenic invaders or can merely compete for the limited nutrients in the digestive tract, thereby preventing them from thriving.
Pathogenic bacteria, on the other hand, benefit while their human hosts suffer, causing anything from mild symptoms, such as pimples in the case of Propionibacterium acnes, to serious symptoms, such as muscle paralysis and even death in the case of Clostridium botulinum. These differing bacterial infections have a broad range of incubation periods and modes of transmission as well, so it’s key that researchers carefully study the pathogens at play when developing measures against them.
Many bacterial outbreaks are the result of contaminated food or unclean surfaces. Prevention of bacterial outbreaks includes strict regulations in the food service industry and rigorous hygiene practices in healthcare environments. Restaurants must be especially
careful when handling food to ensure their customers don’t accidentally ingest infectious agents and find themselves as cases in a potential outbreak.
Important strategies for limiting the spread of pathogenic bacteria include proper storage and preparation of perishable foods, treatment of drinking water, and sufficient personal hygiene. Similarly, all doctors, nurses, and clinicians must wash their hands thoroughly and wear appropriate personal protective equipment –switching their gear between each patient they visit – to prevent the spread of microbes.
Antibiotics are an important tool used to mitigate the proliferation and spread of pathogenic bacteria. We’ll dive into antibiotics and other drugs to treat infectious diseases in Chapter 8: Therapeutics, but for now, just know that antibiotics are used in the treatment of
Neisseria gonorrhoeae
Salmonella typhimurium
Staphylococcus aureus
Streptococcus spp.
Gonorrhea (infects the reproductive and urinary tracts)
Food-borne illness, gastroenteritis (digestive tract infection)
Staph infection, pneumonia, toxic shock syndrome (lungs infection)
Pneumonia, strep throat (lungs infection)
Treponema pallidum Syphilis (infects the skin and internal organs)
bacterial infections. Antibiotics target critical parts of bacteria that prevent them from carrying out their important functions, thereby blocking bacteria from growing in a host and spreading between people. Importantly, antibiotics target key features that bacterial cells have but that human cells lack. While antibiotics have transformed the fight against bacterial infections, the rise of antibiotic resistance – the new inability of previouslyeffective antibiotics to kill the same strain of bacteria – is one of the great public health challenges of our lifetime.
Before antibiotics, bacteria were once the most devastating killers on the planet. The bubonic plague that struck Europe and Asia in the mid1300s, killing tens of millions of people, was
DNA, 2.1 Mb
Gram-negative, diplococci bacteria
DNA, 4.7 Mb
Gram-negative, rod-headed bacteria
DNA, 2.7 Mb
Gram-positive, cocci shaped bacteria that tend to cluster in grape-like structures
DNA, 2.1 Mb
Gram-positive, streptococcus (chains of round cells)
DNA, 1.1 b
Gram-Negative, Spirochaete (spiral shaped)
Note: While some bacteria can have a natural color, the electron micrographs shown in this table have been digitally colorized and do not show the real color of the bacteria. The Neisseria gonorrhoeae image is a medical illustration.
caused by the bacteria Yersinia pestis, which still drives occasional outbreaks today. The sexually transmitted infection syphilis, caused by the bacterium Treponema pallidum, can lead to a slowly progressing disease with devastating physical, neurological, and cardiac symptoms. Today, these and numerous other bacterial diseases are largely curable, thanks to the advent of antibiotics. However, bacterial infections still cause clinical harm, and the threat of antibiotic resistance looms over the global population. In Table 5.2, we provide a few examples of pathogenic bacteria with which we contend today. We’ll then take a closer look at the biology of one important group of bacteria and one of its pathogenic strains affecting humans: Escherichia coli
Escherichia coli
Escherichia coli is a large group of gram-negative bacteria commonly abbreviated as E. coli, and is pictured in Figure 5.10. Bacteria in the E. coli group are one of the most ubiquitous and wellstudied species of bacteria. In humans, it is found naturally in our gut, helping our digestive system maintain proper homeostasis and prevent other pathogens from taking hold in our bodies.
The E. coli genome size varies between species, but on average, it is four million nucleotides (4 Mb) long with roughly three thousand genes. Because E. coli grows very quickly and its genes are well understood, it is one of the most commonly used models for biological research; in fact, much of our understanding of basic biology comes from E. coli-based studies.
While some strains, or specific subtypes, of E. coli are normal inhabitants of our gut microbiome, other strains can cause harm as bacterial pathogens. Has your local news station mentioned an E. coli outbreak recently? They happen fairly frequently, often as a result
FIGURE 5.10 | Cross section of the E. coli cell. In green, we see the cell wall; in magenta and blue, the cytoplasm; in yellow and orange, the nucleoid. E. coli bacteria are naturally found in our gut as part of the microbiome, but some strains are pathogenic and can cause human disease. Image credit: The Machinery of Life by David S. Goodsell, Springer New York, 2009, with permission.
of contaminated food sold at restaurants and grocery stores or poor sanitation practices overall. Pathogenic E. coli can manifest as a urinary tract infection, diarrhea, sepsis, or meningitis—all of which can be life-threatening without proper, timely treatment. These pathogenic strains of E. coli contain virulence factors, which are factors that help them cause and spread disease. Their virulence factors, which are often acquired via plasmids, include compounds that help E. coli evade the immune system, attach to the host tissue, and send out exotoxins, toxins released by bacteria into their surroundings. Based on these virulence factors,
various pathogenic E. coli strains often use similar mechanisms to hide from our immune defenses and multiply. For example, pathogenic E. coli share the ability to bind to mucous membranes to which normal E. coli cannot bind, such as your esophagus, after which they can release toxins and damage host tissue.
One common pathogenic strain of E. coli is Enterohemorrhagic E. coli (EHEC), which can cause severe intestinal infection and is
found naturally in the intestinal tract of cows. Consuming undercooked meat or produce contaminated by cow feces can cause infection in humans, which has led to many foodborne illness outbreaks, including the Chipotle E. coli outbreak in January 2016. EHEC’s main virulence factor is Shiga toxin, which causes damage to the intestinal wall and can result in severe bloody diarrhea, abdominal cramps, nausea, and fatigue.
1. What is the difference between gram-positive and gram-negative bacteria and how is this biological distinction useful to distinguish between types of bacteria?
2. What special feature of the pathogenic bacteria E. coli allows it to spread so quickly within the human body?
3. How can antibiotics help mitigate the spread of pathogenic bacteria?
Protozoa are eukaryotic microorganisms found in most habitats. These microorganisms are very diverse, with over 50,000 species currently known. Protozoa have been found in fossils upwards of 500 million years old. From the Greek words protos and zoon, meaning “primitive, first, or original animals,” early researchers named them for the animal-like behaviors they exhibited, such as motility and predation. Morphologically, they are incredibly diverse and are classified by their means of locomotion; you may be able to spot some when observing pond water under a dissection microscope.
While protozoa are free-living organisms, which means that they are not directly dependent on other organisms for survival, many of them live by siphoning off nutrients from their hosts. In humans, they can be found living in different parts of our bodies, from the gut to the brain. Pathogenic protozoa are parasites, meaning they benefit at the detriment of the host. Their complex life cycles allow them to be spread in many different ways, including through vectors or sexual contact. Humans may also encounter parasitic protozoa in contaminated water or food. The parasite Plasmodium falciparum, causing Malaria, is one of the most well-known, widespread, and deadly protozoan diseases. Still, there is a wide range of other protozoa that cause some of the most prolific infections impacting humans today.
Protozoa are microscopic unicellular eukaryotes. It can be difficult to describe protozoa as a whole since they have incredibly broad characteristics. Protozoan species range greatly in size and shape, as well as reproductive forms. Like other eukaryotes, they have DNA genomes
packed on multiple chromosomes. The smallest reported protozoan genome is 2.3 million bases (2Mb) for the parasite Encephalitozoon intestinalis, and the largest is a whopping 97.8 billion bases (98 Gb) for Gonyaulax polyedra
These eukaryotic microorganisms are extremely complex for a single-celled organism. In addition to the organelles found in most eukaryotic cells, such as the nucleus or ribosomes, some protozoa have special locomotion organelles. These include cilia, pseudopods, or flagellum, which allow the protozoan to move, and a cytostome or cell “mouth” to ingest fluids or solid particles. Protozoa do not have a cell wall, but some have a protein shell called a pellicle that, much like a cell wall, allows them to maintain their structure. A commonly found cell structure of protozoa is pictured in Figure 5.11.
5.11 | Protozoan cellular structure. An illustration of an example of one commonly found protozoa cell structure and its organelles. Protozoa are unicellular microorganisms with a wide variety in size and shape. They are characterized by having additional organelles that other eukaryotic cells don’t have such as a flagellum, and a pellicule labeled.
Most protozoa reproduce asexually via binary fission: similarly to bacteria, protozoa can replicate their DNA and split into two daughter cells. However, some species of protozoa can reproduce sexually, and some can even do both. For example, when Plasmodium
reproduces, they switch between asexual and sexual forms, and some Plasmodium species can undergo different parts of their life cycles within multiple host organisms. The Plasmodium life cycle takes place in two hosts; the parasites first undergo asexual multiplication in the blood cells of the human host and then sexual reproduction in the mosquito midgut (Figure 5.12).
On top of an incredible ability to undergo both sexual and asexual reproduction, protozoan
reproduction is made even more complex by its capacity to switch between dormant and active forms at various life stages.
The parasites take different forms during the different stages of the malaria parasite life cycle. They start as ‘sporozoites,’ spore-like forms, and then become gametocytes, the precursors of the ‘gametes’ or sexual reproduction cells, in the human host. They then transform from gametocytes to ‘sporozoites’ within the mosquito host. SPOROZOITES
FIGURE 5.12 | Life cycle of the malaria causing parasite Plasmodium. The Plasmodium life cycle takes place in two hosts mosquito (left) and human (right) and undergoes multiple stages within each of the hosts. The asexual part of the cycle occurs in the human host and the sexual part in the mosquito. Asexual reproduction: 1) The first step is the transmission to humans: female mosquitoes feeding on human blood transmit ‘sporozoites ,’ the first parasitic form of the malaria parasite. 2) These travel in the bloodstream and infect the liver cells (hepatocytes). Inside the hepatocytes, ‘sporozoites’ replicate and as they increase in number then the liver cells rupture releasing ‘merozoites ,’ a new parasitic form. 3) Merozoites travel in the bloodstream and infect red blood cells (RBCs). RBCs rupture as the parasite develops and duplicates. 4) Mature merozoites develop into male and female ‘gametocytes .’ 5) A mosquito takes a blood meal from an infected human host and ingests the gametocytes. These then travel into the mosquito midgut where they undergo sexual development resulting in ‘gametes .’ Sexual reproduction: 6) female and male parasitic gametes mate and form a ‘zygote’ or ‘egg.’ 7) The zygote continues to develop and penetrates through the mosquito midgut giving rise to thousands of sporozoites.
The active, main stage for all protozoa is called the trophozoite stage, during which they feed and multiply. In the malaria life cycle, the trophozoite stage occurs in the human host. Trophozoites mature into schizonts, which rupture, releasing merozoites. Many protozoa also have a dormant cyst stage, during which they require fewer nutrients and are more resistant to the environment; you can think of this stage as a protozoan “seed” that can spread more easily to others. In the malaria parasite, the cysts – the infectious form of many protozoa – are made in the mosquito host and are known as ‘sporozoites’ with which the infection phase starts.
While protozoa can be free-living, parasitic protozoa derive nutrients from their hosts. These parasites are able to toggle between their life cycle phases, triggering different reactions within their human hosts as a result. For example, the trophozoite stage typically leads to detectable symptoms such as diarrhea and nausea as the protozoa feed on and damage the host. Their dormant cyst stage, during which they develop thickened cell walls and increased resistance to harmful environments, can also further exacerbate their negative effects on the host; the resiliency of this stage allows them to survive exposure to both the host’s immune system and to potential therapeutic treatments. Free-living protozoa cysts can even survive outside of a host, allowing them to move between hosts indirectly; for example, many of the protozoan diseases can be passed through feces, as new human hosts encounter protozoan cysts that have survived passage through an infected individual’s entire digestive tract. These unique strategies can make protozoan diseases especially hard to track, treat, and prevent from spreading to other individuals.
Scan this QR code or click on this link to watch a video about how malaria develops inside a human.
Eukaryotes, protozoa, and humans share cellular similarities, presenting challenges for drug development since the medications that treat the protozoa could also harm the human host cells. Over the years, we have developed several drugs, including chloroquine and artemisinin, which can curb, or even cure, various protozoan diseases without significantly harming the infected individual.
Unfortunately, many strains of protozoa have become resistant to our medications against them. Their quick reproduction rate speeds up the emergence of mutations and thus their ability to adapt to new medications. Despite the challenges, we’ve been remarkably successful at combating various protozoan outbreaks around the world, in large part through sanitation practices. Health professionals have been able to limit the spread of protozoan diseases at their origin by decreasing exposure to potential sources of contamination. For example, outbreaks caused by water-borne protozoan illnesses, such as cryptosporidiosis and giardiasis, which cause diarrhea, have been successfully contained in the past by identifying the source of the contaminated water and employing standard sanitation practices to reduce the risk of transmission. As we discussed in Chapter 2: Epidemiology, the specific modes of transmission certain pathogens often employ can uniquely equip us with tailored tactics in the fight against them.
Parasitic infections caused by protozoa are mainly common in tropical and subtropical areas. Malaria, whose parasite life cycle we’ve already described, is the most deadly parasitic disease in the world, primarily afflicting children in subSaharan Africa. Parasitic infections including Chagas disease, cyclosporiasis, cryptosporidiosis, and giardiasis can be found in all regions, impacting a broad scope of people.
For example, Chagas disease is most common in Latin America in parts of Mexico, Central America, and South America where an estimated 8 million people are infected by the protozoa Trypanosoma cruzi. Chagas disease spreads after a Triatomine bug, aka “kissing bug,” feeds on the blood of a human or animal infected with T. cruzi The newly-infected insect then bites around the eyes or mouth of a human host — the behavior that earned it the “kissing” moniker. When the
Entamoeba histolytica
Giardia lamblia
Trichomonas vaginalis
Trypanosoma brucei
Toxoplasma gondii
Amoebiasis (Intestinal illness)
Giardiasis (intestinal illness)
Trichomoniasis (infects genitals)
African sleeping sickness (central nervous system is affected)
Toxoplasmosis (affects almost any part of the body)
kissing bug eventually poops on a person’s skin, the T. cruzi parasites are released and enter the human host’s body through the fresh bites in the skin. Another significant protozoan illness is the intestinal illness called cyclosporiasis and caused by the parasite Cyclospora cayetanensis, which infects humans after consumption of food or water contaminated with the parasite. Past outbreaks of cyclosporiasis in the US have been linked to imported fresh produce, such as raspberries, basil, snow peas, and mesclun lettuce.
Giardia and Cryptosporidium are the most common protozoan waterborne infections worldwide, causing most of the 251 identified waterborne protozoa outbreaks identified in the US and the UK between 2017 and 2020.
Let’s take a look at other protozoan diseases (Table 5.3). We will then examine the biology of Plasmodium falciparum, the parasite that causes malaria, in more detail.
DNA, 2.4 Mb
DNA, 11.7 Mb
DNA, 160 Mb
DNA, 35 Mb
DNA, 80 Mb
Note: Microscopy images shown in this table are photomicrographs, captured with light microscopes which can magnify objects between 1 to 2000 times their actual size. Typically, microorganisms are stained to facilitate their visualization. The colors seen here may not be representative of their real color. The Giardia lamblia image is a 3D computer recreation based on an electron micrograph.
Endemic to many tropical regions around the world, malaria has been and continues to be, one of the highest-burden infectious diseases in the world. With 200 million cases and 400,000 deaths annually, malaria continues to cause immense harm to humankind. Malaria can be caused by six different species of Plasmodium protozoa , but Plasmodium falciparum is the most devastating culprit. The P. falciparum genome was the first protozoan genome to be sequenced. It is 23 million nucleotides (23 Mb) long and contains 14 different chromosomes with over 5000 genes.
P. falciparum and the other malaria-causing Plasmodium protozoa are carried by a vector, usually Anopheles mosquitoes, to vertebrates like humans. P. falciparum infections cause headaches, nausea, and muscle pain as our bodies fight against the pathogen. In extreme cases, malaria can cause anemia, characterized by a decrease in the number of red blood cells, and respiratory distress, sometimes leading to coma and death.
As we will discuss in Chapter 8: Therapeutics, humans have been trying to mitigate the risks of malaria for centuries. The first known antimalarial, quinine, is believed to have been discovered in the 1600s, in the bark of the cinchona tree. Soon after, it became widely used, and in 1934, researchers discovered chloroquine in the lab, which is structurally similar to quinine. Since then, numerous different antimalarials have been discovered, and malaria control measures focused on reducing the mosquito populations through indoor spraying with insecticides and bed nets have been developed as well.
In 1955, the WHO launched the Global Malaria Eradication Program, powered by their supply of effective antimalarials and insecticides. Their initial success rates led many countries to announce malaria eradication prematurely. Soon after, however, cases rebounded as the parasite and mosquito vectors evolved resistance to existing countermeasures. In 1969, the program stopped, and researchers continue to work towards eradication efforts today.
1. What are some unique biological features of protozoa?
2. How do protozoa reproduce?
3. How do protozoan parasites infect humans?
4. Why is it difficult to fight protozoan infections?
Fungi are a highly diverse kingdom, ranging from the mushrooms you buy in the store to the chytrids – or water molds – that devastate amphibian populations worldwide. The word fungus is directly derived from the Latin word fungus for “mushroom.” Despite this name, there are millions of different fungal species on earth that aren’t just limited to the mushroomshaped ones we’re familiar with. Fungi range from microscopic yeast to macroscopic mushrooms to the largest living organism on earth— the Armirilla bulbosa fungi network in Canyon City, Oregon, US, which covers an area larger than 1,300 football/soccer fields. In this section, we’ll learn more about fungi and how some of them can cause infections in humans.
Similar to protozoa, fungi have a large range of genome sizes across species, varying from less than 10 million nucleotides (10 Mb) to 180 million (180 Mb). Certain fungi can also be multinucleated, having multiple nuclei, in addition to the other organelles that eukaryotic cells have. The majority of fungi cells present a cell wall composed of carbohydrates called chitin and glucans; chitin also makes up the exoskeleton of insects and crustaceans. These cell walls provide the cell with a rigid shape and structure. They also serve as a defense to filter out large toxic molecules and prevent the cell from lysing. Beneath the cell walls, fungi have yet another functional protective layer: a lipid bilayer that serves as a cell membrane, also known as plasma membrane. The unique composition of fungi’s external layers provides researchers with a number of drug targets to treat fungal infections, as we’ll discuss in more detail in Chapter 8: Therapeutics. A commonly found cell structure of fungi is featured in Figure 5.13.
5.13 | Fungus cellular structure. Illustration of a typical cell structure of a fungus, showing a cell wall and eukaryotic organelles.
Fungi have the ability to reproduce sexually or asexually. Asexual reproduction in fungi occurs when cells replicate their components and split to make spores. Like plant seeds or protozoan cysts, spores in fungi can be transported to new areas to find habitats to grow. Importantly, these spores are genetically identical to the original fungus.
Sexual reproduction, however, opens the door for genetic diversification, facilitated by the fusion of special fungal filaments called hyphae. Once these hyphae merge to create one cell, the fused cell splits to create two daughter cells, each with a novel combination of the parent cells’ DNA. These daughter cells are spores that can then be disseminated to form a new hyphae network. While most fungi adhere to the stages of this general life cycle, there are many variations, especially among fungal pathogens.
Although fungal infections are generally perceived as minor in comparison to other microbial infections, fungi can cause illnesses of varying intensity. The severity of these infections
is often largely dependent on the condition of the infected host. In fact, the majority of fungal infections are a result of the opportunistic nature of many fungi. Opportunistic pathogens don’t cause disease under normal conditions but instead cause disease when it is easy for them to do so, i.e., when they have the right opportunity. For example, many people recovering from previous illnesses in hospitals are immunodeficient — meaning that they don’t have a strong enough immune defense system to fend off relatively weaker, less virulent pathogens. We refer to a host as immunocompromised if they are immunodeficient or if there are external factors impairing their immune system, such as various drugs that may inhibit their ability to stop pathogen growth. Therefore, their body becomes an ideal environment for opportunistic pathogens to survive and reproduce.
Treatment of fungal infections – opportunistic or otherwise – can prove difficult. Although mild infections can typically be treated with non-prescription creams and powders like
Table 5.4: Disease-causing Fungi
clotrimazole, which target features specific to fungi such as the fungal cell membrane, newer treatments can be difficult to develop. Since fungi are eukaryotic, they share many common features with human cells. Just as certain protozoa medications could harm the human host as they go after the pathogen, many therapies against fungal infections can disrupt structures within human cells that are similar to the drug’s target. This brings a challenge to the development of therapeutics targeting fungi. We will learn more about it in Chapter 8: Therapeutics.
Although outbreaks caused by fungi are generally overlooked in the world today, fungal infections kill more than 1.5 million people and infect over a billion each year. Perhaps even more worryingly, a small cohort of fungi consistently pose the most significant threats, illuminating our shortcomings in effectively addressing them. Nine-tenths of all deaths due to fungal infection are caused by species of Candida, Aspergillus, Cryptococcus, or Pneumocystis fungi; in Table 5.4, we’ve listed Protozoa
Aspergillus
Candida
Respiratory disease: allergic bronchopulmonary aspergillosis (ABPA) (affects the lungs)
Candidiasis: vaginal yeast infection, oral thrush, skin infection (infects the vagina, the mouth and the skin)
Cryptococcus Cryptococcal meningitis and/or pneumonia (affects the brain and the lungs)
Pneumocystis
Trichophyton
Pneumocystis pneumonia (PCP) (affects the lungs)
Athlete’s foot (infects the skin)
DNA, 38 Mb
DNA, 16 Mb
DNA, 19 Mb
DNA, 8 Mb
DNA, 22.5 Mb
Note: Microscopy images shown in this table are photomicrographs. The microscopic fungi have been stained to facilitate their observation; they do not typically have the colors seen on the images.
these, as well as a few more of the most common fungal infections we currently face. We’ll then take a quick look at two common fungi known to cause disease in humans: Candida albicans and Microsporum canis
As you’ve already read, Candida albicans, a common fungal infection, is normally a commensal fungus found in our bodies. It is especially found in the vaginal microbiome, and is the causative pathogen of the ever-prevalent yeast infection. It is estimated that Candida infections affect up to 3 out of 4 women at some point in their lifetimes. Further, Candida infections are more common after somebody has finished a course of antibiotics because these treatments disturb the microbiome balance by also killing off the protective bacteria and resulting in a weakened microbiome. When this happens, Candida albicans can sometimes take over and cause disease. Additionally, a species of Candida called Candida auris has formed antibiotic resistance and is growing in prevalence, causing outbreaks in hospitals today.
Although ringworm is not a particularly deadly fungal disease, it is a useful example of how fungal infections can behave in the body and spread to other organisms. Ringworm is not a type of worm as its name might imply, but rather a parasitic fungus. It is an infection of the skin and nails that can cause an itchy, discolored, circular rash, hence its name (Figure 5.14). Ringworm can be caused by about 40 different types of fungi, including Microsporum canis, that live either in soil or exclusively on animals. These fungi thrive in moist, warm areas, such as skin folds and athlete locker rooms, latching on to new hosts either through
FIGURE 5.14 | Typical ringworm rash. Ringworm disease can affect different parts of the body, including the feet (known as athlete’s foot) and groin (known as jock itch) and the infections can result in circular rashes. Note that rashes can take on different hues depending on an individual’s skin tone.
contact with fungal matter or through spores on objects such as towels, clothing, footwear, hairbrushes, and exercise equipment. Once they are within the body, the fungi can spread locally at the contact site, disrupting the functions of nearby cells. There are a number of topical antifungal treatments for ringworm, such as ketoconazole, but in more severe cases, oral antifungals may be needed.
1. What characteristic of an individual host may account for a typically-mild fungus causing severe infection?
2. Why are pathogenic fungi particularly challenging to treat?
There is a wide range of infectious agents that cause extensive human disease but are not considered microbes. Some of these can be large living organisms, and others may not be living at all. Here, we’ll go over two key examples that you may come across: helminths and prions.
Helminths, also known as parasitic worms, are multicellular invertebrates with flat, elongated or round bodies causing disease in humans. They have long afflicted human society, with helminth eggs being found in mummified feces that are thousands of years old. The impact of helminths has even shaped modern history. For example, in the Chinese Revolution of 1949, an outbreak of helminth infection in Mao Zedong’s army stopped his invasion of Taiwan long enough for the US to come to the island’s aid.
Helminths are an incredibly varied group of parasites for which effective treatment and mitigation strategies are essential. For instance, helminths are often transmitted due to poor sanitary conditions that result in the unintentional consumption of larvae or eggs. It is estimated that of the approximately 3 billion people worldwide who live on less than 2 US dollars per day, a third are infected by one or more helminths – roughly 1 billion people. This statistic speaks to the ubiquitousness of the issue, as well as the urgency with which it should be addressed, and it further supports our understanding of how various social determinants of health can lead to significant disparities. We will discuss this further in Chapter 10: Social Determinants of Health.
When analyzing the structure of helminths, researchers traditionally characterize these worms into one of two categories: nematodes, also known as roundworms, and platyhelminthes, also known as flatworms. As the names suggest, roundworms are easily identified by their cylindrical shape, in contrast to the flattened bodies of flatworms. Most helminth genomes have been found to fall between 20 million (20 Mb) and 500 million nucleotides (500 Mb) – notably larger than their microbial counterparts. As multicellular, eukaryotic organisms, these organisms tend to be far more complex than microbial infectious agents, and they are often even visible to the naked eye. In fact, nematodes have complete digestive systems, equipped with mouths and body cavities. Platyhelminthes, on the other hand, lack this body cavity and complete digestive system but do have a ‘mouth’ that leads directly to their digestive structures. While neither type of helminth reproduces inside the host organism like other infectious agents, they are able to lay eggs that can either hatch within the host or spread to other environments via the individual’s feces.
Helminth infection most commonly occurs after accidental ingestion of eggs, which are present in the waste of infected individuals. As these eggs can then contaminate soil used to grow crops, these pathogens are referred to as “soiltransmitted.” They ultimately infect individuals who interact with the soil or its agricultural products. Although helminth diseases inhabit a number of body tissues in an infected individual, many of them primarily affect the intestines, causing symptoms such as pain and diarrhea. However, helminth diseases can cause different
symptoms depending on where the worms are localized in the body, such as the lymphatic system – which helps to clear the body of toxins, waste, and other unwanted materials – in the case of lymphatic filariasis. These diseases can also severely impact the overall health of host individuals; as the helminths feed on blood and other tissues, they can cause conditions such as anemia and poor nutrient absorption for sustained periods of time until the infection is treated.
Population-level mitigation strategies for helminth diseases primarily include improving community sanitation and hygiene practices in ways that minimize contact with potential waste or contaminated soil. Helminth diseases are typically endemic to tropical low-income regions, which often have poorer sanitation practices than their wealthier counterparts. Thus, improving sanitation processes can quickly reduce the sources of outbreaks. In addition to community-level interventions, safe and effective treatments for individual patients are also critical. There are several successful anthelmintic medications (meaning medications against helminths) that are employed against common infections today. Unfortunately, the field of anthelmintics development suffers from some of the same issues as other therapeutics, including insufficient research attention and funding, drug resistance, and difficulties in identifying specific drug targets that will not interfere with the cells of the human host. All of these challenges can greatly impede our ability to develop new countermeasures.
Let’s take a closer look at the biology of two common parasitic helminths: Clonorchis and Lymphatic filariasis
Clonorchis is a common parasitic liver flatworm that can be contracted by humans when they eat raw fish or shellfish; in these cases, the fish and shellfish serve as secondary hosts. Liver flatworms infect the liver, gallbladder, and bile ducts of human hosts. Although most infected individuals are asymptomatic, patients with a high parasitic load can experience abdominal pain, nausea, and fever. In extreme cases, Clonorchis infections can give rise to a certain type of cancer and cause chronic irritation. Despite the severe symptoms, there are medications for Clonorchis that can swiftly kill the parasite and treat the infection. These antiparasitics, albendazole, and praziquantel, kill the flatworm or otherwise prevent its proliferation.
Also known as elephantiasis, lymphatic filariasis is a disease caused by the nematodes Wuchereria bancrofti, Brugia timori, and Brugia malayi, referred to as filarial worms. Filarial worms typically infect human hosts via mosquito bites before attacking the body’s lymphatic system. While most cases of lymphatic filariasis are largely asymptomatic, these patients can still experience kidney damage, as well as harm to their lymphatic and immune systems as their bodies become less effective at flushing out toxins. Patients that do experience severe disease, however, are forced to endure physically and socially disruptive symptoms, including thickened skin, as well as severe swelling of the arms, legs, breasts, or genitals (Figure 5.15). In addition to these difficulties, these individuals often must deal with immense social stigma, and the disease’s physical deformities are generally permanent.
We now have fairly effective treatment protocols, which involve community-level treatment with
FIGURE 5.15 | Patient presenting lymphatic filariasis (elephantiasis) symptoms. Bodily deformities associated with lymphatic filariasis add to the stigma of those infected.
common antiparasitics such as albendazole and ivermectin, that can prevent individuals in particularly high-risk regions from contracting lymphatic filariasis. Despite this, the disease remains shockingly prevalent in the world today. In 2018, 51 million people were reported to be infected, and while this number reflects a substantial decline from previous years, it still reflects the disease’s status as a neglected tropical disease – a disease that disproportionately lacks attention and robust public health response due to its limited prevalence outside of the developing world.
Another key example of a non-microbial infectious agent is the prion. Prions are abnormal misfolded proteins that can aggregate (clustered together) and accumulate in infected tissue causing tissue damage and disease (Figure 5.16). Prions are always microscopic in size; in fact, they are even smaller than viruses. However, they differ from every other infectious agent discussed in one crucial way: they do not have the characteristics of a living pathogen
and do not replicate in the way a virus would within a host cell. While all eukaryotes, prokaryotes, and even viruses are composed of various macromolecules including proteins, a prion is a molecule, a protein, in and of itself.
Unlike multicellular organisms, prions do not require any metabolic or digestive functions to survive. As misfolded proteins, they have no genetic material or organelles. Although prions don’t traditionally “reproduce” (another reason for not being considered living), they do cause infections by causing other nearby proteins to similarly misfold.
Prions are typically passed between organisms through direct contact with food and bodily fluids or indirectly from contaminated meat and the environment. After infection, prions generally are found in brain tissue. They are not visible to the naked eye, making them difficult to identify until after an infected individual dies and their brain can be examined.
FIGURE 5.16 | Prions. Misfolded proteins can lead to protein aggregation, causing disease. Here, we present a model of normal and disease-causing proteins. The different colors in the prions represent different protein domains – regions in proteins that fold independently providing a specific structure on proteins known as loops (blue), beta-sheets (pink), and alpha-helices (yellow).
Prions cause a class of fatal disorders called transmissible spongiform encephalopathies (TSEs), in which misfolded proteins accumulate in the brain and interfere with neurological function. TSEs always impact the nervous system, quickly and fatally disrupting these essential tissues. In fact, most deaths following prion infection occur within a few months.
While prions may initially seem like minor threats due to their nonliving status, they are often some of the most arduous infectious agents to eliminate. Because they are proteins rather than organisms with unique biological structures, developing drugs that successfully target and distinguish them from normal proteins in the human body is challenging. Additionally, they cannot be degraded at the temperatures we typically use to sterilize objects; while most bacteria can be killed at temperatures higher than 65 °C (150 °F), certain prions can remain stable at temperatures exceeding 200 °C (392 °F) without denaturing or degrading. Since these prions are found in the brain and spinal cord tissue of infected organisms and can rarely jump across species, they are encountered relatively infrequently. Given their persistence, this is good for susceptible organisms like us. Due to this infrequency, primary mitigation strategies are
primarily targeted toward the food industry, regulating the treatment of cattle and meat preparation to ensure that no contaminated food is sold.
One highly publicized outbreak of a prion disease occurred in the United Kingdom (UK) in 1986 when young people began unexpectedly coming down with a variant of CreutzfeldtJakob disease (CJD), a human TSE. The origin of this outbreak was eventually traced to a disease in cows, Bovine Spongiform Encephalopathy (BSE), nicknamed “Mad Cow Disease’’ because of its neurological effects. It was determined that the cows had been infected with prions, which were then passed to humans through the consumption of affected meat. Despite the fact that cases of BSE peaked in the UK nearly 30 years ago, it remains an ongoing public health concern and may even be heritable. It is suspected that 1 in every 2,000 UK residents is a carrier of BSE, an alarmingly high prevalence rate for a disease with the potential genetic transmission. As such, the FDA has dictated guidelines for blood and other tissue donations from individuals who spent extended time in the UK during the BSE outbreak.
1. How are helminths usually transmitted?
2. What are prions, and why are they especially difficult to detect?
1. Microbiology studies microscopic organisms and their biology.
2. Scientists discovered that microscopic organisms were the cause of disease as they investigated the blood of plague victims under a microscope. They observed microorganisms and confirmed they were the cause of the disease by following Koch’s postulates.
3. Koch’s postulates state
a. The microorganism must be only found in individuals with the disease and not in healthy individuals.
b. The microorganism isolated from the individuals with the disease must be cultured (grown in the lab under sterile and controlled conditions).
c. It must be possible for a healthy individual who is inoculated with the cultured microorganism to fall ill with the disease.
d. It must be possible to isolate the microorganism from a newlyinfected individual, and the isolated microorganism must match the original microorganism.
4. Viruses, bacteria, protozoa, fungi, parasitic worms (helminths) and prions.
1. Viruses are considered non-living because they are acellular (not made up of cells) and they cannot reproduce without using the replication machinery of a host cell.
2. Integration shelters the virus and allows the virus to stay dormant in a way that it can “hide” from the immune system.
3. Integrated viruses are more difficult to treat than lytic viruses because they become part of host DNA, and are therefore difficult to target without harming the host.
4. During a viral infection’s incubation period, the virus’s primary task is to replicate at very high rates within the infected individual.
1. Gram-positive bacteria only have two layers in their cell walls—the rigid, peptidoglycan cell wall and the inner membrane. Gram-negative bacteria cell walls contain three layers: an outer membrane, an intervening cell wall, and an inner (cytoplasmic) membrane. Gram stain can be used to visually identify grampositive bacteria from gram-negative bacteria, allowing researchers to narrow down the list of bacteria they might be looking at.
2. E. coli replicates very quickly, with a new generation being produced about every 20 minutes; for comparison, our fastest replicating human cells replicate only every few weeks.
3. Antibiotics target critical parts of bacteria that prevent them from carrying out their important functions, thereby blocking pathogenic bacteria from growing in a host and spreading between people.
1. Protozoa are single-celled eukaryotic microorganisms. They are diverse in their structure and reproduction but mostly divide through binary fission. Unique biological features of protozoa include cilia, flagellum, and even cytosomes (cell ‘mouths’).
2. Most protozoa reproduce asexually via binary fission: similar to bacteria, protozoa can replicate their DNA and asexually split into two daughter cells. However, some species of protozoa can reproduce sexually, and some can even do both.
3. They can be spread in many different ways, including through vectors, water and food, and sexual contact.
4. Protozoa and humans share many cellular similarities, making protozoa difficult to target when developing treatments since the medications that treat the protozoa could also harm the human host. Many strains of these pathogens have become resistant to existing medications due to the high speed of asexual reproduction and a high mutation rate, speeding up adaptation to selective pressures in the environment.
1. The individual may be immunocompromised, allowing the pathogen to “take advantage” of their weakened immune system and causing them to have more severe symptoms.
2. Since fungi are eukaryotic, they share many common features with human cells. Certain medications could harm the human host as they go after the pathogen. Many therapies against fungal infections can disrupt structures within human cells that are similar to the drug’s target.
1. Helminths are often transmitted due to poor sanitary conditions that result in the unintentional consumption of larvae or eggs.
2. Prions are misfolded proteins rather than living organisms that can cause devastating effects in nearly all hosts. They are particularly hard to detect because they are not visible to the naked eye, and it is very hard to identify them in brain tissue until the host has died.
Acid-Fast Bacteria: Bacteria resistant to decolorization by acids during laboratory staining procedures due to their chemical composition. Notable examples are the Mycobacterium species responsible for tuberculosis and leprosy.
Anemia: A health condition marked by low red blood cell count; it can result from malaria caused by the parasite Plasmodium
Antibiotic: A medicine that either kills or inhibits the growth of bacteria.
Antibiotic Resistance: The inability of previously-effective antibiotics to kill the same strain of bacteria.
Asexual Reproduction: A type of reproduction in which there is no union of gametes; the offspring that arise are genetically identical to the singleparent organism.
Bacteriology: The branch of biology studying bacteria.
Binary Fission: A type of asexual reproduction where a cell grows to twice its size, duplicates its genetic material, and then splits into two daughter cells; reproduction is a method typical of bacteria.
Cell Wall: Cellular structure surrounding the plasma membrane found in plants, fungi, and bacteria cells.
Chitin: Carbohydrate that forms part of the fungal cell wall; also makes up the exoskeleton of insects and crustaceans.
Commensal: A biological relationship between organisms where one organism benefits from another without causing it any harm.
Cyst: The infectious form of many protozoa presenting a protective membrane or thickened wall.
Cyst Stage: The life stage of protozoa during which they require fewer nutrients and are more resistant to their environment; also known as the protozoan ‘seed’ stage.
Exotoxin: Toxins released by bacteria into their surrounding environment.
Flagella: A tail-like structure that provides motility for some microorganisms.
Fungi: Eukaryotic organisms that have complex life cycles and perform extracellular digestion; range from single-celled to highly-complex multicellular eukaryotic organisms particularly dangerous to immunocompromised individuals.
Glucans: Macromolecules composed of multiple sugars.
Gram-Negative Bacteria: Bacterial cells whose walls contain three layers: an outer membrane (consisting of lipopolysaccharides), an intervening cell wall, and an inner (cytoplasmic) membrane. Inner and outer membranes are semi-permeable, meaning they only allow certain molecules to pass through. Appear pink under the microscope when stained with the Gram stain.
Gram-Positive Bacteria: Bacterial cells that due to their composition retain the Gram stain and appear violet under the microscope.
Gram Stain: A staining method for classifying bacteria based on their cell wall composition.
Helminths: Parasitic worms, multicellular invertebrates, or spine-lacking eukaryotes with elongated bodies that have long afflicted humanity.
Immunocompromised: A term to refer to people whose immune systems are weakened or compromised because they are immunodeficient, or because of some other external factor.
Immunodeficient: People who do not have enough immune defenses to fend off even weaker, less virulent pathogens; their bodies are ideal environments for opportunistic pathogens.
Incubation Period: The number of days between a microbe infecting a host and when the host shows symptoms, ranging from just one day to several months.
Koch’s Postulates: A set of criteria formalized by Robert Koch that helped to determine whether or not a specific microbe was the cause of a specific disease. The four criteria state:
1. The microorganism must be only found in individuals with the disease and not in healthy individuals.
2. The microorganism isolated from the individuals with the disease must be cultured (grown in the lab under sterile and controlled conditions).
3. It must be possible for a healthy individual who is inoculated with the cultured microorganism to fall ill with the disease.
4. It must be possible to isolate the microorganism from a newly-infected individual, and the isolated microorganism must match the original microorganism.
Lipopolysaccharides (LPS) : Large molecules consisting of a lipid and polysaccharide that are found exclusively on the outer membrane of Gram-negative bacteria.
Lysis : Rupture of cells as a consequence of the breaking down of their cell membranes.
Lysogenic Cycle : The form of viral reproduction in which the viral genome integrates into the host’s genome, allowing it to lie dormant within the cell until a signal tells it to activate and switch to the lytic cycle.
Lytic Cycle : The form of viral reproduction in which the virus uses the host’s cellular machinery to make several copies of itself and ultimately lyse the cell.
Microbiology : The scientific study of microscopic organisms.
Microbiome : A diverse community of microorganisms in your body; that plays important roles in your body’s natural functions (e.g., breaking down food into nutrients).
Mutualistic : A biological relationship where both parties (e.g., bacteria and host) benefit.
Nematode: A subdivision of helminths, also known as roundworms, are elongated cylindrical parasitic worms, including hookworms and whipworms.
Non-Microbial Infectious Agents: Infectious agents are not classified as microbes due to their differing size or molecular nature, which can still cause severe human diseases. Nonmicrobial infectious agents include helminths and prions.
Opportunistic Pathogens: Pathogens that don’t cause disease under normal conditions, but can cause disease when it’s easy for them to do so. They often take advantage of individuals with compromised immune systems, manifesting in these individuals with a greater severity than their infections typically would.
Parasites: Invading organisms that benefit from the host at the host’s detriment.
Pellicle: A protein shell similar to a cell wall that coats some protozoa and allows them to maintain their structure.
Peptidoglycan: A complex sugar-and-protein molecule that forms the bacterial cell wall, acting as an exoskeleton (external skeleton) and allowing bacteria to maintain structure in conditions where they might otherwise lyse.
Pili: Tube-like structures on some bacteria that allow for the passage of genetic material to other nearby bacteria that aren’t necessarily related; like bacterial “straws” that allow them to share DNA with one another.
Plasmid: A genetic structure in a bacterial cell that can replicate independently of the chromosomes; typically a small circular DNA strand in the cytoplasm of a bacterium.
Platyhelminthes: Subdivision of helminths, also known as flatworms, are worms with a soft, usually flattened body; includes flukes and tapeworms.
Prion: A type of non-microbial infectious agent; misfolded or aggregated protein that
causes surrounding normal proteins to misfold or aggregate as well that can cause disease in humans and spread.
Protozoa: A diverse group of single-celled eukaryotes with complex life cycles and unique cellular structures, among which include several widespread parasitic organisms (e.g., Plasmodium).
Quarantine: The restriction of movement to individuals suspected to have been exposed to contagious diseases in order to prevent spread.
Spores: Specialized single cells that allow fungi to reproduce. Also the term for hardy structures that some bacteria produce when they are under adverse conditions.
Transmissible Spongiform Encephalopathies: A class of fatal disorders caused by prions in which misfolded proteins accumulate in the brain and interfere with brain function.
Trophozoite: The main stage of life for protozoa, during which they feed and multiply.
Viral Latency: The ability of a virus to remain inside a cell dormant without being active for long periods of time.
Virology: The study of viruses and their biology.
Virulence Factors: Cellular structures and molecules that help pathogens achieve colonization of a niche in a host, hide from the host’s immune system, weaken the host’s immune system, or take nutrients from the host.