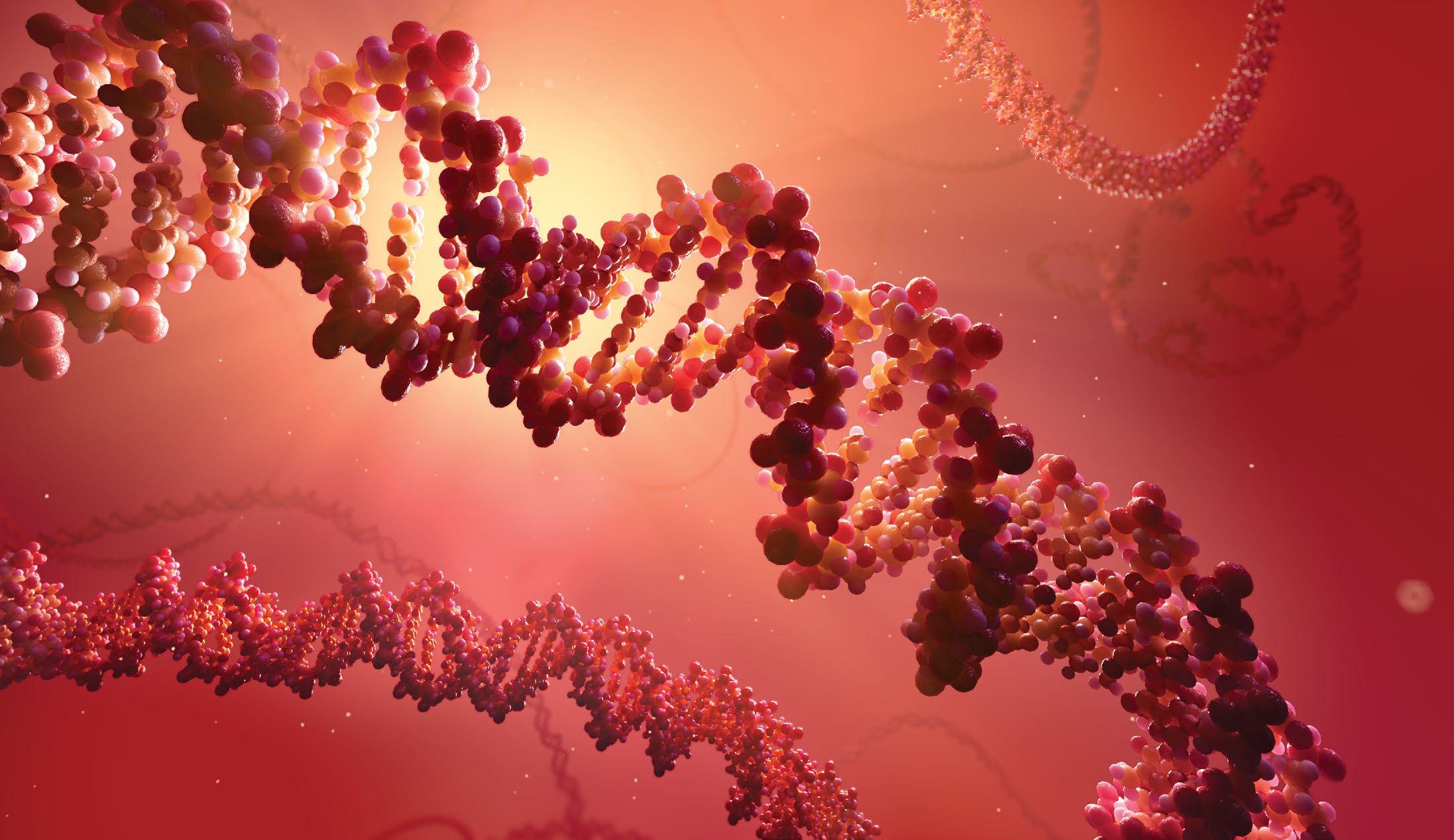
13 minute read
What Is Epigenetics and How Is It Relevant for Spine Surgeons?
Differential epigenomic markers have been associated with a number of spinal, musculoskeletal, and inflammatory conditions.[1-3] Recently, epigenetic research has garnered considerable attention due to the multidirectional relationship between environmental exposures, genetic variables, epigenetic variables, and health/disease conditions. While the excitement has created considerable interest and hope for potential applications of this research, many are unaware of both the potential promises and pitfalls of epigenetic research. In this article, we introduce epigenetics as a concept, discuss challenges of epigenomic research, and apply this discussion to critically engage with research on osteoarticular diseases.
Understanding Epigenetics
The idea of an epigenomic landscape is not new. Waddington proposed the term epigenotype in the 1940s. Nanney subsequently reinterpreted epigenetics to include cellular memory in the 1950s, and Riggs and Holliday related DNA methylation with epigenetics in the 1970s.[4] Recent technological advancements have allowed epigenetic studies to flourish, and the applications of epigenetics for spine health and disease are just starting to be explored.
A basic understanding of epigenetics often starts by breaking down the etymology of the term: epi, which means over or beyond. As such, epigenetics is often described as above and beyond the genome. Epigenetics is more specifically defined as “the study of mitotically and/or meiotically heritable changes in gene function that cannot be explained by changes in DNA sequence.”[5] The boundaries of genomic control that are encompassed within epigenetics are debated, but the mechanisms typically discussed are gene methylation, histone modification, and noncoding RNA.
Each of these epigenetic mechanisms affect genomic activity. We classically have taught about DNA as a fixed sequence— the nucleotide sequences an individual is born with generally does not change over an individual’s lifespan. We also often first introduce genomic sequences in a rather deterministic manner—this nucleotide sequence predicts this outcome. Studying the epigenomic landscape has the potential to shift this immutable and deterministic view as we learn more about the ways in which epigenetic mechanisms affect genetic activity and impact physiology, as well as how these mechanisms work in multidirectional ways, with relationships explored between genomic, epigenomic, phenotypic, and environmental variables.[6,7]
One of the keys to understanding epigenomics is to consider accessibility and readability of nucleotide sequences. Within the nucleus, DNA is compactly wrapped around histones. The more densely packed the DNA and histone proteins, the less likely transcription will occur. The more loosely packed, the more accessible it is for transcription. Enzymatic changes to histones with acetyl, methyl, or phosphate groups modify this accessibility, thereby affecting the potential transcription of specific regions of DNA.[8]
Methyl groups can also be added to DNA nucleotides. Methylation typically occurs to cytosine nucleotide positionally followed by a guanine (CpG). This is an enzymatic process that can be reversed, meaning methyl groups can be added or removed. Methylation at CpG islands (clusters of CpG dinucleotides located near gene promoter regions) is typically associated with gene silencing. While early research focused on DNA methylation at CpG islands, more contemporary work takes whole genome approaches, including intragenic regions, CpG islands, CpG shores, and CpG valleys.[9]
Noncoding RNA (ncRNA) represent the majority of transcribed material.[10] Some of what was once described as junk DNA is now known to produce ncRNA with regulatory and structural functions. Regulatory ncRNA often explored in epigenetics research include microRNAs (miRNAs), which are short ncRNAs typically 20–24 nucleotides in length, and long non-coding RNAs (lncRNAs), which are ncRNAs typically greater than 200 nucleotides in length. These ncRNAs can play a role in expression regulation at both the genetic and chromosomal level.[10,11]
In this article, we could repeat the sentence “differential epigenomic markers are associated with [insert spinal condition here],” as differential epigenomic markers have been associated with osteoarthritis, osteoporosis, ankylosing spondylitis, bone mineral density, spinal cord injury, adolescent idiopathic scoliosis, tumorous subtypes, aging, and more.[1-3,12-23] However, the existence of an association only tells us so much. Thus, in the rest of this article, we will first discuss challenges of epigenomic research necessary for spine surgeons to be able to better critique the research they encounter. We follow this with a synthesis of data regarding osteoarticular diseases with a particular focus on applying these critiques.
Challenges in Epigenomic Research
Epigenetic mechanisms respond to and influence phenotypes and environmental variables, which is why epigenetic mechanisms are often seen as possible mechanistic links between exposures and health outcomes. There are nuances within epigenetic research that are critical to be aware of when interpreting the promises of this research.
Heijmans and Mill reviewed cautionary warnings for clinical epigenomics relevant for a nuanced understanding of epigenomic research results. They first emphasized that researchers often look first at gene methyl- ation, rather than histone modification or non-coding RNA, while each mechanism may play a critical role in health and disease.[24] Additionally, similar to genome-wide association studies, epigenetic studies are identifying small differences in a number of CpG sites.[24] The meaning of methylated state for specific cytosines still needs to be explored; the existence of differential methylation tells us only part of the story. Learning what methylation or absence of methylation at each specific site signals for transcription will help fill in gaps. [24] Furthermore, while we have made strides in technological advancements, our tools are still imperfect.[9,24] Sample size and timing is a critical issue for mechanisms known to change over time and hypothesized to change in relation to health, disease, environment, treatments, etc.[2,24] Additionally, there is tissue-specificity in epigenetic markers, so the correlation may be low between the tissue of interest and the tissue available to measure (most often blood or saliva).
Existing biases in research, such as lack of diverse populations, sampling methods, and the misperceptions and biases of scientists affecting study design, cannot be ignored in epigenetic work. Just as in nongenomic epidemiological research, 25 studies have shown that scientists’ assumptions about sex, gender, sexuality, class, and social disparities can affect epigenetic research design and interpretation.[26] Randomized clinical trials in spine surgery are under scrunty for a lack of diverse representation,[27] which is an issue that should be scrutinized in spinal epigenetic research, too.
Finally, multiple scholars warn that the high expectations and popular interest in medical epigenomics create a certain amount of hype that epigenetics may not be able to fully realize.[9,24]
Epigenetics and Osteoarticular Diseases: Osteoarthritis, Osteoporosis, and Ankylosing Spondylitis
Epigenetic mechanisms have been implicated in osteoarticular diseases including osteoarthritis, osteoporosis, and ankylosing spondylitis.[1-3] The site specificity observed in osteoarticular diseases has given rise to the hypothesis that epigenetic modifications may act as a mechanistic link between patients’ underlying genes and intrapersonal heterogenous diseases.[1]
One critique of epigenetic studies is the focus on DNA methylation in research, but epigenetic studies on osteoarthritis, osteoporosis, and ankylosing spondylitis have included research on DNA methylation, histone modification, and ncRNA activity.[1,2] A related critique focuses on the meaning of epigenetic states: are these epigenetic states correlated with changes in gene expression or cellular physiology? In one study, articular cartilage sampled from the femoral head showcased differential DNA methylation between patients with and without osteoarthritis, and the differential methylation was correlated with changes in gene expression.[16] A separate study reported that levels of a histone methyltransferase (SET-1A) increase in osteoarthritic cartilage and that the activity of this histone methyltransferase increases expression of genes correlated with loss of chondrocytes and inhibition of collagen production.[1,15]
Similarly, differences in DNA methylation, histone acetylase and deacetylase activity, and miRNA expression have been observed in patients with ankylosing spondylitis compared to healthy controls.[18-20] For example, one study compared methylation of a gene SOCS-1 in serum of 43 patients with ankylosing spondylitis to six control patients, reporting that methylation of SOCS-1 correlated with cytokine activity and severity of ankylosing spondylitis disease.[18] Furthermore, a number of studies have reported a correlation between ncRNA and ankylosing spondylitis, specifically observing that miRNAs associated with ankylosing spondylitis have functional targets involved in regulation of T cell survival and bone remodeling (eg, osteoblast proliferation or osteoclasts activation).[2] In epigenetic research on osteoarticular diseases, work is being conducted on multiple epigenetic mechanisms, and the potential meaning of these differential epigenetic states for gene expression and cellular physiology is being explored.
Another critique of epigenomic research in general relates to its reliance on imperfect samples and sample sizes—a critique that can be readily applied to epigenomic research of osteoarticular conditions. Whole blood, cartilage, and bone samples have all been used to identify differential epigenetic markers associated with either osteoarthritis or osteoporosis.[1,14,16] Whole blood, peripheral blood mononuclear cells, and T cells, have all been used to identify differential epigenetic markers associated with ankylosing spondy- litis.[1,2] Between these different sample types, there are differences in which epigenomic markers are correlated with osteoarticular conditions. Furthermore, most epigenetic work regarding osteoarthritis involves knee and hip studies rather than studies of the spine. Differences exist in epigenetic markers between similar tissues sampled from the hip and knee[1]; thus, there may be unique epigenetic markers associated with osteoarthritis and osteoporosis in the spine.
Further challenges in epigenomic research exist regarding the timing of sampling. DNA methylation is a stable marker, but it is also responsive to the environment. This means these epigenetic markers may change prior to disease onset, over the course of disease progression, or in response to treatments. Sampling pertinent tissue from humans across osteoarticular disease progression is unethical.[28] Yang et al also raise the possibility that treatments for ankylosing spondylitis such as nonsteroidal anti-inflammatory drugs (NSAIDs) also affect DNA methylation.[2] Studies comparing control patients to patients with ankylosing spondylitis need to address these potential confounding factors.
A final critique verges on managing the high expectations of epigenomic research promises. In osteoarticular conditions, more research is needed to fully understand the complex relationships observed. While therapeutic potentials have been explored, they show no benefit to osteoarthritis thus far.[1] Roberts et al suggested that the most promising use for epigenetic markers is as a biomarker of disease.[1] Understanding epigenetic changes over the course of osteoarticular disease progression may aid in staging the disease.[1]
Future Directions
It is an exciting time for the -omics world as technological breakthroughs allow for more efficient and less expensive sequencing. In this article, we reviewed some of the ways we should be critical of epigenomic studies in conditions affecting the spine. Specifically, we encourage understanding of the many epigenomic mechanisms and multidirectional relationships between genomic, epigenomic, condition, and environmental variables. We caution on the tissue specificity of epigenetic markers, epigenomic changes over the course of disease, and the potentially confounding variables that create challenges for designing sound research methodology. While more research is needed, there are promising applications of epigenetic research for the spine. Epigenetic research holds promise for risk screening, diagnostic screening, disease staging, and potential prognoses.[29] The use of epigenetic biomarkers is being explored to aid in the prognosis of spinal cord injury[21] and adolescent idiopathic scoliosis.[12]
Tumor subtypes are sometimes classified by DNA methylation patterns, such as in Ewing’s sarcoma, in which methylation patterns are associated with tumor aggression.[23]
As more epigenomic research studies are conducted, it is important to be aware of the challenges inherent in epigenomic research. This will allow us to improve study designs, critique existing research, and analyze possible applications of epigenetic research related to the spine.
References
1. Roberts SB, Wootton E, De Ferrari L, Albagha OM, Salter DM. Epigenetics of osteoarticular diseases: recent developments. Rheumatol Int . 2015;35(8):1293-305.
2. Yang H, Chen Y, Xu W, et al. Epigenetics of ankylosing spondylitis: Recent developments. Int J Rheum Dis . 2021;24(4):487-493.
3. Altorok N, Nagaraja V, Kahaleh B. Chapter 14: Epigenetics in bone and joint disorders. In: Tollefsbol TO, ed. Medical Epigenetics. 2nd ed. Academic Press; 2021:251-278.
4. Greally JM. A user’s guide to the ambiguous word ‘epigenetics’. Nat Rev Mol Cell Biol. 2018;19(4):207-208.
5. Russo VEA, Martienssen RA, Riggs AD. Epigenetic Mechanisms of Gene Regulation. Cold Spring Harbor Laboratory Press; 1996.
6. Non AL, Thayer ZM. Chapter 19: Epigenetics and human variation. In: O’Rourke ZM, ed. A Companion to Anthropological Genetics . Wiley; 2019:293-308.
7. Lappé M, Landecker H. How the genome got a life span. New Genet Soc . 2015;34(2):152-176.
8. Fischle W, Wang Y, Allis CD. Histone and chromatin cross-talk. Curr Opin Cell Biol. 2003;15(2):172-183.
9. Non AL, Thayer ZM. Epigenetics for anthropologists: an introduction to methods. Am J Hum Biol. 2015;27(3):295-303.
10. Kaikkonen MU, Lam MT, Glass CK. Non-coding RNAs as regulators of gene expression and epigenetics. Cardiovasc Res . 2011;90(3):430-440.
11. Wei JW, Huang K, Yang C, Kang CS. Non-coding RNAs as regulators in epigenetics [review]. Oncol Rep. 2017;37(1):3-9.
12. Perez-Machado G, Berenguer-Pascual E, Bovea-Marco M, et al. From genetics to epigenetics to unravel the etiology of adolescent idiopathic scoliosis. Bone . 2020;140:115563.
13. Horvath S, Raj K. DNA methylation-based biomarkers and the epigenetic clock theory of ageing. Nat Rev Genet . 2018;19(6):371-384.
14. Delgado-Calle J, Fernandez AF, Sainz J, et al. Genome-wide profiling of bone reveals differentially methylated regions in osteoporosis and osteoarthritis. Arthritis Rheum. 2013;65(1):197-205.
15. El Mansouri FE, Chabane N, Zayed N, et al. Contribution of H3K4 methylation by SET-1A to interleukin-1-induced cyclooxygenase 2 and inducible nitric oxide synthase expression in human osteoarthritis chondrocytes. Arthritis Rheum. 2011;63(1):168-179.
16. Kim KI, Park YS, Im GI. Changes in the epigenetic status of the SOX-9 promoter in human osteoarthritic cartilage. J Bone Miner Res. 2013;28(5):1050-1060.
17. Swingler TE, Wheeler G, Carmont V, et al. The expression and function of microRNAs in chondrogenesis and osteoarthritis. Arthritis Rheum. 2012;64(6):1909-1919.
18. Lai NS, Chou JL, Chen GC, Liu SQ, Lu MC, Chan MW. Association between cytokines and methylation of SOCS-1 in serum of patients with ankylosing spondylitis. Mol Biol Rep. 2014;41(6):3773-3780.
19. Lai NS, Yu HC, Chen HC, Yu CL, Huang HB, Lu MC. Aberrant expression of microRNAs in T cells from patients with ankylosing spondylitis contributes to the immunopathogenesis. Clin Exp Immunol. 2013;173(1):47-57.
20. Toussirot E, Abbas W, Khan KA, et al. Imbalance between HAT and HDAC activities in the PBMCs of patients with ankylosing spondylitis or rheumatoid arthritis and influence of HDAC inhibitors on TNF alpha production. PLoS One . 2013;8(8):e70939.
21. Zhang BY, Chang PY, Zhu QS, Zhu YH, Saijilafu. Decoding epigenetic codes: new frontiers in exploring recovery from spinal cord injury. Neural Regen Res . 2020;15(9):1613-1622.
22. Morris JA, Tsai PC, Joehanes R, et al. Epigenome-wide Association of DNA Methylation in Whole Blood With Bone Mineral Density. J Bone Miner Res . 2017;32(8):1644-1650.
23. Fatema K, Luelling S, Kirkham M, Pavek A, Heyneman AL, Barrott J. Chapter 6: Epigenetics and precision medicine in bone and soft tissue sarcomas. In: García-Giménez JL, ed. Epigenetics in Precision Medicine Academic Press; 2022:147-191.
24. Heijmans BT, Mill J. The seven plagues of epigenetic epidemiology [commentary]. Int J Epidemiol. 2012;41(1):74-78.
25. van Ryn M, Saha S. Exploring unconscious bias in disparities research and medical education. JAMA . 2011;306(9):995-996.
26. Kenney M, Müller R. Of Rats and Women: Narratives of Motherhood in Environmental Epigenetics. In: Meloni M, Cromby J, Fitzgerald D, Lloyd S, eds. The Palgrave Handbook of Biology and Society. Palgrave Macmillan UK; 2018:799-830.
27. Issa TZ, Lambrechts MJ, Canseco JA, et al. Reporting demographics in randomized control trials in spine surgery - we must do better [advance online publication November 17, 2022]. Spine J. doi:10.1016/j.spinee.2022.11.011
28. Housman G, Quillen EE, Stone AC. An evolutionary perspective of DNA methylation patterns in skeletal tissues using a baboon model of osteoarthritis. J Orthop Res . 2021;39(10):2260-2269.
29. Chaves P, Onieva JL, Barragán I. Chapter 7 - Epigenetic biomarkers of disease. In: Tollefsbol TO, ed. Medical Epigenetics. 2nd ed. Academic Press; 2021:117-141.