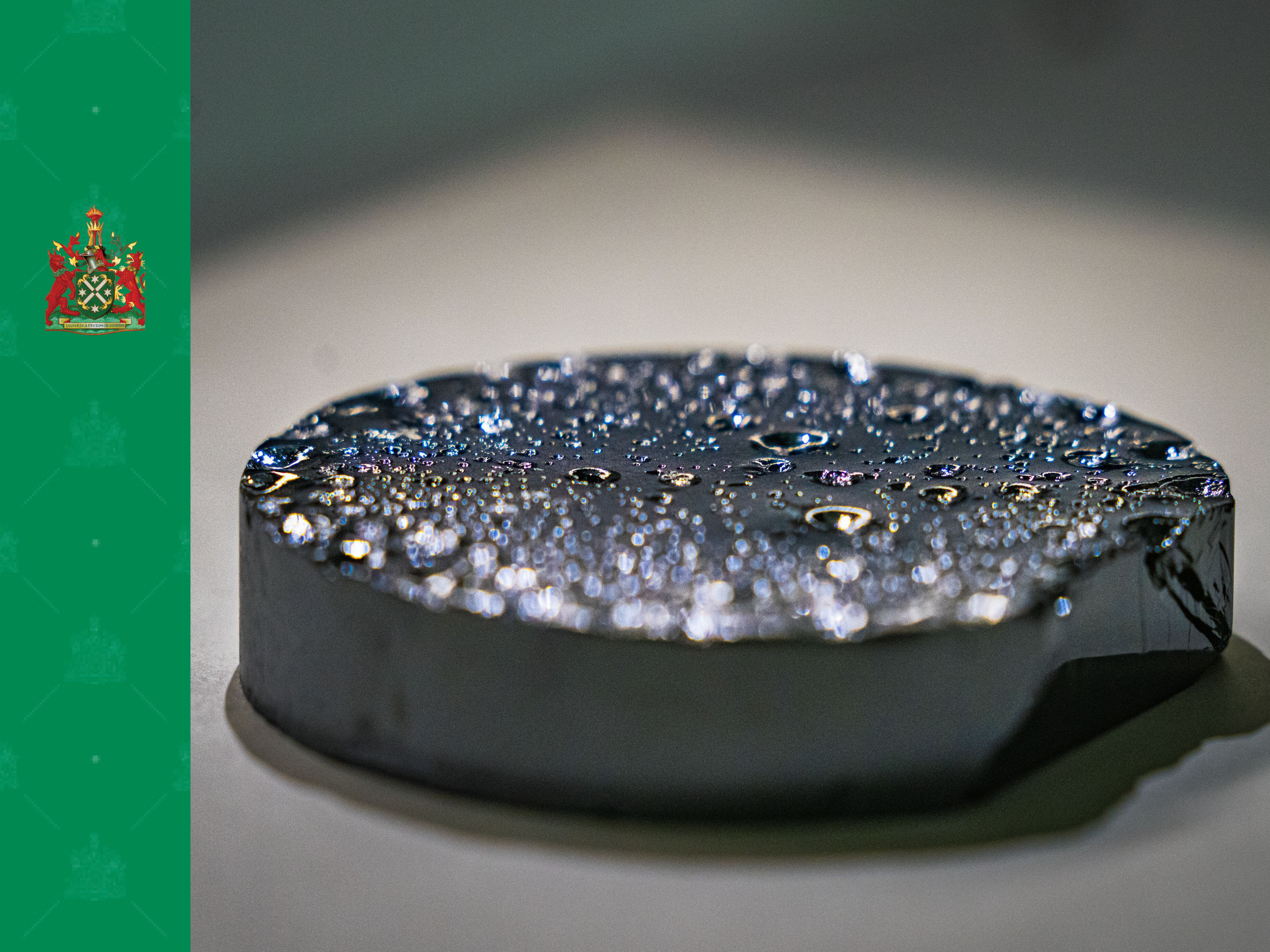
15 minute read
Photon-Counting CT: A Leap from Black & White TV to Colour HDTV
from Inside News September 2022
by RANZCR

Dr Christian Schroeter
Photon-counting computed tomography (PCCT) promises to revolutionise CT imaging by substantially increasing spatial resolution and iodine contrast conspicuity, reducing radiation dose and beam hardening artifacts, and allowing spectral imaging with multiple energy bins.
Unlike traditional energy integrating detectors which use solid-state scintillators to first convert X-ray photons to light, photon-counting detectors use a semiconductor material to directly convert incoming X-rays to an electric signal in a single step. Each photon gives rise to a voltage that is proportional to its energy, allowing energy discrimination on the basis of voltage comparison. A count can therefore be assigned to the bin that matches the photon’s energy range, hence the name “photon-counting”. With enough bins, one can therefore perform spectral analysis of the incoming photon flux, and extract spectral information for quantitation or material decomposition. Since lower energy photons, which produce more contrast information, get equal weighting to higher energy ones, the images have improved contrast resolution.
Prof Roland Bammer, Head of Monash University’s Department of Imaging*, describes this transition. “Remember the times when you were watching sports on a low-res black and white TV with the “bunny ears” on top? Now you can watch events in colour on your 4K TV. This is the paradigm shift in CT that’s currently happening with photon-counting. The ‘colour’ depth isn’t OLED quality yet, and more like on the old CGA or VGA monitors, but it’s there. You can do all the interesting things energy integrators can’t do without dual kV source or kV switching. PCCT does the energy discrimination on the detector side, and opens up a new field in radiology.”
Although it has long been known that photon counting is the most efficient approach to X-ray detection for CT, developing a direct conversion material which can handle the high X-ray photon flux of CT has been the major challenge. Prof Bammer says
Physicist Dr Christian Schroeter, PhD, who led the effort to develop the direct conversion detector material for Siemens Healthineers, talks to Associate Professor Shalini Amukotuwa, PhD, MB BS, FRANZCR (Head of Diagnostic Neuroradiology and MSK at Monash Health) about the detector and his experience in developing it.
Photon-counting detectors have brought about a paradigm shift in CT imaging. Can you tell us what the main advantages of this technology are, compared to the conventional CT scanners which we are currently using?
Improvement in these crucial technical parameters translates to clinical benefits, which we have been working on since the prototype phase. We have already seen that there are certain things that you can only do with a photon-counting CT. For example, a really hot topic is imaging stented coronary arteries. The metallic stents cause a lot of artifacts, due to beam hardening and photon starvation, which obscure the vessel lumen. These patients are therefore often sent to cathlab instead of CT coronary angiography. Now, with photon-counting CT, we can get really good images of the vessel lumen, to see in-stent details. This is because of the far superior spatial resolution, reduced blooming and beam hardening, and lack of electronic noise, which we can filter out. The spectral information also allows us to obtain material (iodine) images of the vessel lumen, and we can use material decomposition to subtract out any calcified plaques. We are currently exploring whether these images are now accurate enough for catheter angio to be avoided, which will be a big game changer for patients. Another important example is imaging obese patients, and low-dose imaging, where photon counting provides better image quality with less noise because electronic noise is eliminated. The journey has only just begun, and we will continue to see what these technical advantages really mean in clinical practice, since that’s what’s important.
Why did it take so long to develop photon-counting CT scanners? What was the main barrier to overcome?
The direct conversion material for the detector was the main barrier, and we had a long development phase. The project started 20 years ago. We seriously engaged in screening the market of available detector materials around 15 years ago. The first phase was to look at what was already available, then select the best vendor. We identified a company in Japan which made cadmium telluride (CdTe) detectors. But the detector still wasn’t good enough for CT, to be able to deal with the high X-ray photon flux. We had to work on the properties of the material to make it CT ready. It was a tremendous challenge around 2008/9 to overcome this basic hurdle of the detector response to the X-ray photons.
What were the properties in particular that you had to develop that existing detector materials didn’t have?
The main challenge at that time, 15 years ago, was that most manufacturers used the detector material for gamma cameras, for SPECT, or just spectrometers. The company that we selected already used CdTe detector material for X-ray imaging, although this has a much lower X-ray photon flux than CT. Of all imaging applications, CT has by far the highest X-ray photon flux applied to the detector. The materials which were available then just couldn’t cope with this high flux. There were even scientific papers claiming, based on models, that it may not be possible for CdTe detectors to convert X-rays to photons directly at the typical flux-rates of CT. In the initial prototypes, we could slow down the flux to address this. However, for a clinical full body scanner, you need to be able to work at high flux rates, to be able to deal with any clinical scenario. We were lucky that we came to a point, around 2011, where we could overcome this apparent fundamental hurdle and major impediment which otherwise would have put an end to the project. Another group did actually give up on photon counting using cadmium zinc telluride (CZT) at this time.
Are you are referring to polarisation, which is a property of the material whereby very high X-ray photon flux rates paralyse the detector and reduce its response? What was the solution?
Yes. The solution was based in the CdTe crystal growth itself. You have to go very deep into the very start of the process chain, to the crystal itself, to address the problem of polarisation. You need to understand the whole chain, from crystal growth to image generation, and we saw that many aspects of the crystal growth, for example doping (where tiny amounts of impurities are introduced to alter the crystal structure), the temperature treatment, and the electrodes that we applied, needed to match the bulk material. We had to bring all these pieces together, and understand the signal that comes out of the detector material to design the electronics, the application specific integrated circuit, accordingly. But the trick was really in the first steps, and it was quite a basic one.
So those steps reduced the amount of charge (hole) trapping in the detector material, which is the cause of depolarisation?
Yes. The assumptions, which were made in the models which showed that building a CT detector from CdTe would not be possible, were not as simple as initially thought. The models that we now have, based on our empiric observations and better understanding of the detector material, are quite different. It turns out that hole trapping is not such an issue after all! In fact, it never was a fundamental issue for CdTe, but back then, we didn’t have the knowledge and understanding to have this insight and confidence. Now, the fact that there is a clinical whole-body photon-counting CT shows that the argument that CdTe can’t be used as a detector just doesn’t hold true. We have also seen that there is another company in Canada which is stepping into prototyping at a clinical level using a detector with similar material, CZT. I’m therefore confident that they have also overcome this basic hurdle.
Can silicon detectors work for photon-counting CT?
Spatial resolution is the major weakness of silicon-based detectors, and also radiation dose efficiency because of lower absorption which is due to the lower atomic number. With any detector material, you can, with the right system design, bring out one specific parameter and excel at it. For example, extremely high spatial resolution, energy resolution, or dose efficiency. That is nice for a scientific paper or a prototype, but not for a clinical product. You need to have a holistic approach, and bring together and harmonise these parameters for a clinical scanner. You also need scan speed, which is underestimated, and not just for cardiac applications. Dose efficiency and spatial resolution maybe the most important, but then speed, and then energy information. We worked on this integration and harmonisation for 10 years, starting with prototypes scanners and ending with the final product. In my understanding and firm opinion, silicon just can’t do this.
Do you need more than four energy bins?
We will see. It felt like the end of the journey when we launched the clinical scanner, now it seems like it’s just the beginning. We will see what the clinicians and researchers will find out. But right now, in my opinion, even if you have one threshold, you already have most of the advantages of photon-counting, like dose efficiency, high spatial resolution, and absence of electronic noise. If you have two energy bins, you can do iodine separation. With a third bin, then you can do crazy stuff, with new contrast agents such as gadolinium and gold particles, which have a k-edge. It is possible then to make an additional energy separation, but this is a very early, pre-development research topic. The scanner has a fourth bin, so you are future proof in case somebody invents something where you need it. More bins than that are superfluous.
You had a pivotal role in the development of this new technology as the project lead for direct conversion materials (the CdTe detector material). Can you describe your work and that of your team?
I’ve worked through different roles. I initially started out as a detector physicist, straight out of my PhD in 2007, with a focus on the CdTe material itself. My job was to measure and model the material, determine why we didn’t have the signal from the detector that was needed for CT, find out what signal was needed for CT, and then identify what property it was inside the crystal that prevented us from getting the response that we needed. Then, I had to discuss these findings with my colleagues in Japan to determine what we could change to get the properties that we needed for photon-counting CT. That was the first phase, at the end of which (in 2014) we had proof with a pre-clinical whole-body prototype CT scanner. These were assessed at three clinical sites, and we proved that clinical CT image quality was achievable for any body part. We then turned our attention to industrialising the processes. In a prototype, you can hand-build, handtune, exchange and refine components until it works. For serious commercial production, on the other hand, you need reproducibility and quality in your process, as well as scalability. In this second phase, the German and Japanese teams joined forces, to bring our German process engineering and quality skills, and combine them with the fundamental knowledge of crystal growth that our Japanese partners had. Bringing this together wasn’t easy, but we did a really good job of building a highly effective inter-cultural team. It allowed us to ramp up production. Now, in phase 3, we have a fully developed clinical scanner. The task at hand is to see what makes clinicians happy. To ask: what does the PCCT allow them to do that can’t be done with classical CT technology? And what are the differentiators? This brings us back to the first question that you asked.
So how many years of research and development went into this, from the start of phase 1 to when you went live with the first commercially available clinical, whole-body PCCT?
15 years. Even after the detector material is sorted, there is a long journey from a lab prototype to a preclinical prototype, then clinical prototypes, preproduction and finally serious production. You need to walk through each of these stages. This road takes longer for the pioneers than for those who follow, but they still need to go through these stages. Therefore, if we consider the question of silicon which you mentioned earlier, it is a new technology, and you still need to go through these stages. If you are only starting to scan humans, you can easily estimate from the timelines that we had how long it takes to have a product.
Was there a eureka moment for you and your team?
Overcoming the scientific barrier was a pivotal moment. You have such a huge parameters space to play with, with the crystal growth, the electrodes, and what you do with the detector response, with your readout electronics. Most of the things that we tried out in crystal growth had either no effect or a negative one. But once we found the parameter that really improved things, then we dug deeper, and it helped us to understand. Even the failures did help us to understand and model crystal growth. I remember this moment when we had the right parameter to crack the polarisation challenge in our hands, and then it got clearer and clearer that photon-counting CT will be feasible.
That was a little over 10 years, when, within one year, we made the transition from “maybe not feasible” to “will be feasible”. This was a really exciting time.
How long does it actually take to grow the CdTe crystals, it sounds like a very complex process with well-orchestrated steps?
It takes three months for the actual crystal growth step in our method, the travelling heater method (THM). But it actually takes longer, because THM is a two-step approach, with two kinds of crystal growth consecutively. First, we react the cadmium telluride to form a polycrystal that we then use as the feed stock in the second step THM. It’s very complicated. From filling the starting materials, cadmium and telluride, into an ampoule until we get to the finished crystal takes 6–8 months. Then there are further processing steps: slicing, polishing, photolithography to apply the electronics, making the module, and making the detector.
So, what’s next in PCCT? Will there be other scanners using this detector?
We have to distinguish between the first clinical photon-counting CT product, and our photon-counting detector. The basic technology is an element inside the detectors. This will not change anytime soon, since there is very small potential to improve it in a way that radiologists would even notice. What we are working on now are applications. What do we do with the large amount of data coming out of the scanner? How do we bring this data to the user, so that they can make the best use of what the machine produces for clinical impact? We will focus on that, and provide applications and training to really harvest all the advantages that the technology offers.
* Prof Bammer is the Co-founder, Board Director and CTO of RapidAI.
Associate Professor Shalini Amukotuwa
Head of Diagnostic Neuroradiology and MSK at Monash Health