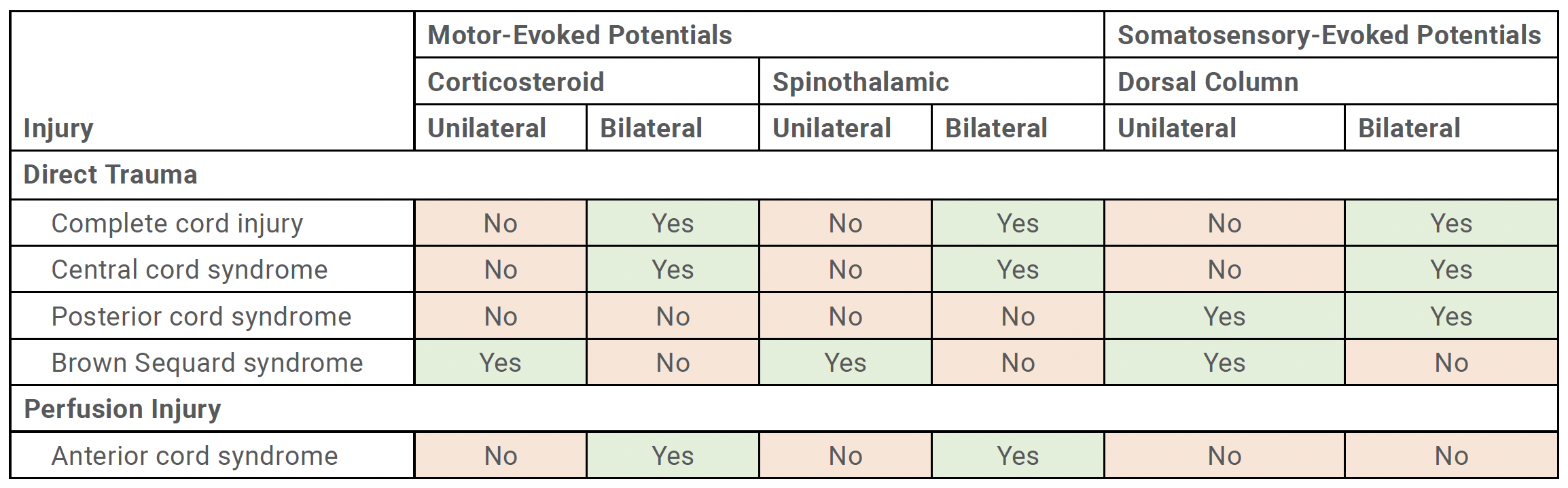
12 minute read
Understanding and Managing Intraoperative Neuromonitoring Changes in Spinal Deformity Surgery
Preventing neurologic complications during spinal deformity surgery is a fundamental objective for spine surgeons. Despite ongoing advancements in surgical techniques and technology, the incidence of intraoperative neurologic injury can reach up to 23% in complex deformity cases.1-3 These injuries can have profound consequences, significantly diminishing a patient’s quality of life, increasing the likelihood of secondary health problems, and creating substantial challenges for both the patient and their family. As a result, intraoperative neuromonitoring (IONM) has become a standard practice in spinal deformity surgeries.4 To take full advantage of IONM, surgeons should be aware of the various IONM modalities, learn the correlation between IONM changes and injury patterns, and integrate systematic protocols to address IONM events.
IONM Modalities
Somatosensory-Evoked Potentials
Somatosensory-evoked potentials (SSEPs) monitor the integrity of the dorsal column of the spinal cord. As such, this modality is useful for monitoring during laminectomies, intradural tumor resections, sublaminar wire insertion, and hook implant insertions. SSEPs measure fine touch and proprioception, but not pain, temperature, or motor function. Therefore, paralysis or significant postoperative motor deficits can occur despite preserved SSEPs.5 Halogenated anesthetic agents, nitrous oxide, hypothermia, and intraoperative hypotension may also affect SSEP readings.6 The warning criteria for SSEPs includes a decrease of more than 50% in amplitude or an increase of more than 10% in latency of signals.
Motor-Evoked Potentials
Motor-evoked potentials (MEPs) reflect the function of the anterior column of the spinal cord, specifically the integrity of descending motor pathways along the corticospinal tract and spinothalamic tract. This modality is particularly useful for measuring spinal cord perfusion/ischemia (anterior spinal artery), insult from pedicle screw constructions, traction, and deformity correction. The stimulation site for transcranial MEP (tcMEP) is the cerebral cortex via subdermal electrodes or magnetic tcMEP. Subdermal electrodes can cause scalp edema and occasional unreliable recordings but are preferable given their low impedance and secure positioning in the scalps. Endpoint data are recorded from either the spinal cord (D-wave) or from end muscle compound motoraction potentials (CMAP). The CMAP is best monitored at sites rich in corticospinal tract innervation, such as distal limb muscles. These commonly include abductor pollicis brevis, adductor hallucis brevis, vastus, and tibialis anterior. The warning criteria for tcMEP includes a decrease of more than 65% to 80% amplitude in the CMAP; however, this threshold currently varies between institutions.6-8 It is important to note that short-acting neuromuscular blockade should be used during intubation. Therefore, total intravenous anesthesia (TIVA) is preferred for this modality.
Electromyography
Electromyography (EMG) applications include both spontaneous EMG (sEMG) recordings and high stimulus triggered EMG (tEMG). In the former, subdermal needles are placed in myotomes that are preselected to coordinate with operative levels. Continuous electrical activity to a myotome may be recorded and provide real-time feedback on possible nerve root irritation. Bursts of activity may indicate possible nerve root impingement, whereas a train of activity is indicative of a more severe root irritation. Complete loss of electrical activity generally correlates with a nerve root being cleanly severed. Muscle relaxants must not be given due to their potential to suppress or dampen all activity.
tEMG can provide information on the integrity of a cortical wall along the transpedicular tract through which a screw has been passed. Bone has a high impedance that requires a high threshold to stimulate an adjacent nerve. Therefore, if the tEMG requires a higher degree of stimulation, the pedicle cortex is likely intact. Lenke et al determined the intensity threshold for safe pedicle screw placement in the lumbar spine with the use of triggered EMG using animal models with clinical correlations.9 Pedicle screws with stimuli intensities larger than 8 milliamperes (mA) were found to be entirely within the pedicle. Those with intensities between 4 and 8 mA demonstrated a potential medial pedicle wall defect. Those with intensities less than 4 mA had a strong likelihood of medial pedicle wall defect and potential contact with neurologic structures. In a retrospective study of 4,857 lumbar pedicle screws, Raynor et al concluded the probability of detecting a medial pedicle breach in the lumbar spine increases with decreasing stimuli thresholds of triggered EMGs.10 In their study, the medial breach rate for screws that required a stimulation greater than 8 mA was 0.31%. For those with thresholds between 4-8 mA, <4 mA, and <2.8 mA, the breach rate was 17.4%, 54.2%, and 100%, respectively. In the thoracic spine, the triggered EMG threshold results vary, but generally thresholds less than 6 mA were indicative of a medial pedicle screw breach. Triggered EMG values lower than these thresholds warrant further tactile and radiographic evaluation.
Stagnara Wake-Up Test
The Stagnara wake-up test was the original form of intraoperative spinal cord monitoring and continues to be used as a means of confirming gross neurologic function. It provides a gross assessment of the primary motor cortex and anterior motor pathways, but it does not provide information on sensory pathways or individual nerve roots. In this test, temporary reduction of anesthesia is required while assessing upper and lower extremity movement. If properly administered, the wake-up test can be extremely accurate in detecting gross motor changes and alert the surgeon of the most clinically significant neurologic deficits, including paraparesis, paraplegia, and foot drop. Known risks associated with this test include self-extubation, postoperative recall of the event, loss of intravenous access, and air embolism. The major limitation of this test is that it is entirely reliant on patient compliance and cannot be used in patients who cannot follow commands, such as infants, those with cognitive or developmental disabilities, or those with preexisting extremity weakness. Another limitation is that it tests the patient’s neurologic status at a single moment in time, and neurologic changes may still occur after the patients are placed back under anesthesia. The intraoperative wake-up test is also used in patients with unobtainable spinal cord monitoring data and in those in whom the IONM has reached warning criteria without improvement after corrective measures.
Correlating IONM Changes With Mechanism of Injury
Recognizing the pattern of IONM data loss is critical to understanding the type of spinal cord injury and potential mechanism of injury. Spinal cord injury can be caused by a multitude of factors, which can be categorized into either direct trauma or spinal cord ischemia (Table 1).
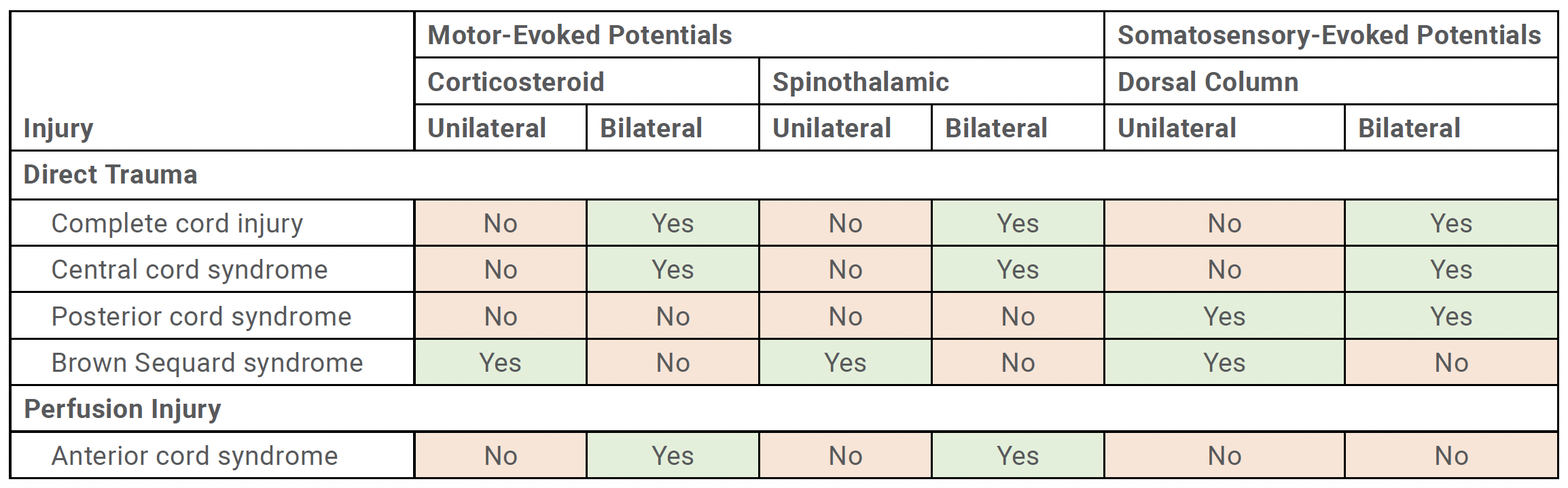
Direct Trauma
Direct trauma includes misplaced implants, impingement of cord, or iatrogenic insult by an instrument. This may result in either complete cord injury, central cord syndrome, posterior cord syndrome, or Brown Sequard syndrome. When bilateral MEP and bilateral SSEP meet warning criteria, this suggests that there is a complete cord injury or central cord syndrome. This typically involves bilateral corticospinal tracts, spinothalamic tracts, and dorsal columns. Bilateral loss in SSEP with preservation of MEPs suggests a posterior cord syndrome. Unilateral MEP data loss and unilateral SSEP loss typically indicates a Brown Sequard to confirm that they are in their appropriate positions. The proper position of the patient’s neck and limbs on the operative table should be confirmed as well.
Second, confirm adequate spinal cord perfusion, which may involve elevating mean arterial pressure (>90 mm Hg) and optimizing hematocrit. In a retrospective analysis of more than 12,000 spinal surgeries involving both adults and children, inadequate spinal cord perfusion emerged as the second most frequent cause of intraoperative neuromonitoring alerts.13 This issue accounted for roughly 12% of all alerts, following screw malposition, which was responsible for 30%. In a study by Lee et al, a drop in hematocrit (Hct) of ≥12 points from preoperative to intraoperative levels was a significant risk factor for IONM data loss.14 Interestingly, the volume of transfusion and estimated blood loss did not emerge as key variables. This finding implies that patients with higher preoperative Hct should be evaluated for transfusion if a substantial decline in Hct occurs, even when intraoperative Hct levels remain relatively elevated.
Third, identify the pattern of IONM loss in the context of the actions or events preceding data loss. For example, if there is loss in bilateral MEPs without changes in SSEPs in the setting of a corrective maneuver, then this suggests an anterior cord perfusion-based injury. The corrective maneuver should be released, and spinal cord perfusion should be optimized. Reassessment of neuromonitoring data should be performed after each intervention. If there is improvement of IONM signals after the release of correction, the surgeon should consider a more moderate correction versus in situ fusion with minimal correction after spinal cord perfusion is optimized. If no improvement in IONM is observed, other causes of spinal cord compression or compromise should be contemplated. For instance, osteotomy sites should be inspected before and after corrective maneuvers. The relationship between bony structures and neural elements may change after deformity correction, and areas of the spinal cord, cauda equine, or nerve roots that initially seemed free may now be compressed by overhanging lamina, pedicles, or other neighboring structures. If there is no improvement after these actions, consider other modalities such as descending neurogenic evoked potentials, wake-up test, or potentially a staged procedure.
Implications of IONM Data Improvement
IONM not only provides greater clarity for when IONM data loss occurs but also can reassure surgeons that data recovery after an intraoperative intervention indicates a reduced risk for neurologic deficit. In a recent study of 1,106 pediatric and adult spinal deformity patients, Lee et al reported an overall rate of cord-level IONM loss in 4.8% of patients and postoperative neurologic deficit in 1.6% of patients.15 The discrepancy between IONM loss and actual postoperative motor deficits was attributed to the recovery of IONM signals observed in 85% of cases after intraoperative interventions. Notably, all patients with IONM data loss underwent intraoperative corrective measures. Improvement in IONM data, whether partial (improvement out of warning criteria) or complete (baseline values), was significantly associated with a reduced risk of postoperative day (POD) 1 motor deficits, with absolute risk reductions of 59% and 89%, respectively. Conversely, patients whose IONM signals did not recover by closure uniformly developed new motor deficits on POD1. Moreover, by the time of discharge, all but 1 patient (88%) without IONM improvement continued to exhibit motor deficits relative to their preoperative baseline. This highlights the critical role of intraoperative intervention and IONM recovery in mitigating postoperative neurologic complications.
Conclusion
Managing IONM loss requires a structured and systematic approach to ensure timely identification of the underlying causes and appropriate intervention. By collaborating with the neuromonitoring and anesthesia teams to rule out technical factors, optimizing spinal cord perfusion, and carefully reassessing surgical maneuvers and anatomy, surgeons can significantly mitigate the risk of permanent neurologic deficits. The recovery of IONM signals following intraoperative interventions serves as a crucial predictor of favorable postoperative outcomes, emphasizing the value of prompt and effective management. These strategies, supported by established best practice guidelines, underscore the importance of IONM in safeguarding neurological function during complex spinal deformity surgeries.
References
1. Lenke LG, Fehlings MG, Shaffrey CI, et al. Neurologic outcomes of complex adult spinal deformity surgery: results of the prospective, multicenter Scoli-RISK-1 study. Spine (Phila Pa 1976). 2016;41(3):204-12.
2. Fehlings MG, Kato S, Lenke LG, et al. Incidence and risk factors of postoperative neurologic decline after complex adult spinal deformity surgery: results of the Scoli-RISK-1 study. Spine J. 2018;18(10):1733-1740.
3. Kato S, Fehlings MG, Lewis SJ, et al. An analysis of the incidence and outcomes of major versus minor neurological decline after complex adult spinal deformity surgery: a subanalysis of Scoli-RISK-1 study. Spine (Phila Pa 1976). 2018;43(13):905-912.
4. Halsey MF, Myung KS, Ghag A, et al. Neurophysiological monitoring of spinal cord function during spinal deformity surgery: 2020 SRS neuromonitoring information statement. Spine Deform. 2020;8(4):591-596.
5. Deletis V, Sala F. Intraoperative neurophysiological monitoring of the spinal cord during spinal cord and spine surgery: a review focus on the corticospinal tracts. Clin Neurophysiol. 2008;119(2):248-264.
6. Devlin VJ, Schwartz DM. Intraoperative neurophysiologic monitoring during spinal surgery. J Am Acad Orthop Surg. 2007;15(9):549-560.
7. Hilibrand AS, Schwartz DM, Sethuraman V, et al. Comparison of transcranial electric motor and somatosensory evoked potential monitoring during cervical spine surgery. J Bone Joint Surg Am. 2004;86(6):1248-1253.
8. Schwartz DM, Auerbach JD, Dormans JP, et al. Neurophysiological detection of impending spinal cord injury during scoliosis surgery. J Bone Joint Surg Am. 2007;89(11):2440-2449.
9. Lenke LG, Padberg AM, Russo MH, Bridwell KH, Gelb DE. Triggered electromyographic threshold for accuracy of pedicle screw placement. An animal model and clinical correlation. Spine (Phila Pa 1976). 1995;20(14):1585-1591.
10. Raynor BL, Lenke LG, Bridwell KH, Taylor BA, Padberg AM. Correlation between low triggered electromyographic thresholds and lumbar pedicle screw malposition: analysis of 4857 screws. Spine (Phila Pa 1976). 2007;32(24):2673-2678.
11. Vitale MG, Skaggs DL, Pace GI, et al. Best practices in intraoperative neuromonitoring in spine deformity surgery: development of an intraoperative checklist to optimize response. Spine Deform. 2014;2(5):333-339.
12. Lenke LG, Fano AN, Iyer RR, et al. Development of consensus-based best practice guidelines for response to intraoperative neuromonitoring events in high-risk spinal deformity surgery. Spine Deform. 2022;10(4):745-761.
13. Raynor BL, Bright JD, Lenke LG, et al. Significant change or loss of intraoperative monitoring data: a 25-year experience in 12,375 spinal surgeries. Spine (Phila Pa 1976). 2013;38(2):E101-E108.
14. Lee NJ, Lenke LG, Arvind V, et al. A novel preoperative scoring system to accurately predict cord-level intraoperative neuromonitoring data loss during spinal deformity surgery: a machine-learning approach [published online November 20, 2024]. J Bone Joint Surg Am. https:// doi.org/10.2106/JBJS.24.00386
15. Lee NJ, Lenke LG, Yeary M, et al. Does an improvement in cord-level intraoperative neuromonitoring data lead to a reduced risk for postoperative neurologic deficit in spine deformity surgery? Spine Deform. 2025;13(1):261-272.
Contributor:
Nathan J. Lee, MD
From the Department of Orthopedic Surgery at Midwest Orthopedics at Rush in Chicago, Illinois.