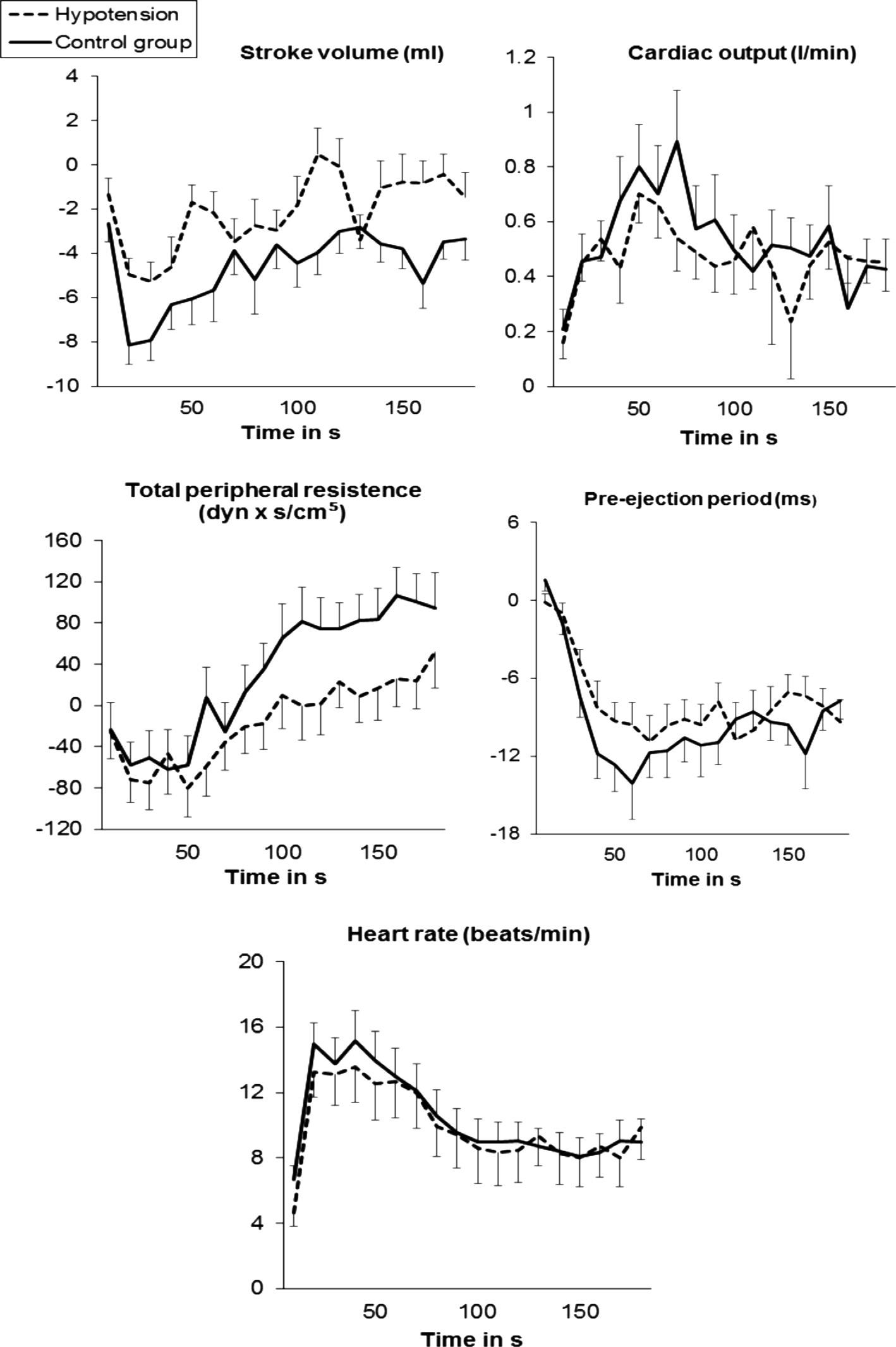
51 minute read
Autonomic Cardiovascular Dysregulation at Rest and During Stress in Chronically Low Blood Pressure Stefan Duschek, Alexandra Hoffmann, Casandra I. Montoro, and Gustavo A. Reyes del Paso
Autonomic Cardiovascular Dysregulation at Rest and During Stress in Chronically Low Blood Pressure
Stefan Duschek, 1
Advertisement
Alexandra Hoffmann, 1
Casandra I. Montoro, 1 and Gustavo A. Reyes del Paso 2
1
UMIT –University of Health Sciences Medical informatics and Technology, Institute of Psychology, Hall in Tirol, Austria 2
Department of Psychology, University of Jaén, Spain
Abstract: Chronic low blood pressure (hypotension) is accompanied by symptoms such as fatigue, reduced drive, faintness, dizziness, cold limbs, and concentration difficulties. The study explored the involvement of aberrances in autonomic cardiovascular control in the origin of this condition. In 40 hypotensive and 40 normotensive subjects, impedance cardiography, electrocardiography, and continuous blood pressure recordings were performed at rest and during stress induced by mental calculation. Parameters of cardiac sympathetic control (i.e., stroke volume, cardiac output, pre-ejection period, total peripheral resistance), parasympathetic control (i.e., heart rate variability), and baroreflex function (i.e., baroreflex sensitivity) were obtained. The hypotensive group exhibited markedly lower stroke volume, heart rate, and cardiac output, as well as higher pre-ejection period and baroreflex sensitivity than the control group. Hypotension was furthermore associated with a smaller blood pressure response during stress. No group differences arose in total peripheral resistance and heart rate variability. While reduced beta-adrenergic myocardial drive seems to constitute the principal feature of the autonomic impairment that characterizes chronic hypotension, baroreflex-related mechanisms may also contribute to this state. Insufficient organ perfusion due to reduced cardiac output and deficient cardiovascular adjustment to situational requirements may be involved in the manifestation of bodily and mental symptoms.
Keywords: hypotension, blood pressure, autonomic control, baroreflex, heart rate variability, cardiac output
Introduction
The term chronic hypotension refers to a persistent state of inappropriately low blood pressure independent of the occurrence of further pathological conditions (De Buyzere, Clement, & Duprez, 1998). According to WHO (1978) criteria, hypotension is diagnosed when systolic blood pressure falls below 100 mmHg in women and 110 mmHg in men. The chronic form is distinguished from orthostatic hypotension (i.e., circulatory problems when assuming an upright position) and symptomatic hypotension, which occurs, for example, due to blood loss or medication (Freeman et al., 2011). The prevalence of chronic hypotension has been estimated at 2–3% in the general population with women being predominantly affected (Pemberton, 1989). In contrast to elevated blood pressure, chronic hypotension is commonly not regarded as a dangerous medical condition requiring treatment (De Buyzere et al., 1998). While hypertension
constitutes a major risk factor for cardiovascular diseases, low blood pressure is associated with reduced cardiovascular mortality (Prospective Studies Collaboration, 2002). Nonetheless, affected individuals frequently report complaints including fatigue, reduced drive, dizziness, headaches, and cold limbs (Pilgrim, 1994; Rosengren, Tibblin, & Wilhelmsen, 1993; Wessely, Nickson, & Cox, 1990). A number of studies furthermore demonstrated cognitive impairment in affected individuals, particularly in the fields of attention and memory, which has been ascribed to suboptimal cerebral blood flow regulation and blunted cortical activity (Duschek, Meinhardt, & Schandry, 2006; Duschek & Schandry, 2004, 2006; Duschek, Weisz, & Schandry, 2003). Aberrances in autonomic cardiovascular control were hypothesized to be involved in the etiology of chronic hypotension (Covassin, de Zambotti, Cellini, Sarlo, & Stegagno, 2013). Previous data pointed toward a reduction
in cardiac sympathetic outflow in hypotensive versus normotensive subjects (Duschek, Heiss, et al., 2009). The estimation of hemodynamic parameters from continuous blood pressure recording (“Modelflow analysis”; Wesseling, Jansen, Settels, & Schreuder, 1993) revealed diminished stroke volume (SV) and cardiac output (CO) in a hypotensive sample at rest and under conditions of mental stress. Considering the beta-adrenergic innervation of the ventricles, the finding suggests lower cardiac contractility due to diminished beta-adrenergic activity (Berntson, Quigley, Norman, & Lozano, 2016; Levy & Pappano, 2007). As a restriction, it should be noted that the reduction in CO –that is given by the product of SV and heart rate –was partly due to lower heart rate, which underlies both sympathetic and parasympathetic control. In addition, the precision of the Modelflow technique in hemodynamic recording is certainly limited (Bogert & Lieshout, 2005). Another line of research defined characteristics of autonomic cardiovascular control in hypotension during sleep. Impedance cardiography revealed lower nocturnal CO and higher values of pre-ejection period (PEP) and left ventricular ejection time (LVET) in affected individuals (Covassin, de Zambotti, Cellini, Sarlo, & Stegagno, 2012; de Zambotti, Covassin, Cellini, Sarlo, Torre, et al., 2012). While LVET cannot be unambiguously related to the sympathetic system, PEP is inversely related to beta-adrenergic activity; as such, its increase is consistent with the notion of reduced myocardial sympathetic outflow (Cacioppo et al., 1994; Hassan & Turner, 1983). By definition, the findings obtained during sleep are not necessarily generalizable to the waking state.
Increased parasympathetic tone may also contribute to persistently low blood pressure (Duschek & Schandry, 2007). Vagal cardiac influence is commonly quantified by means of heart rate variability (HRV) analysis (Berntson et al., 2016). Consistent with the present assumption, hypotensive individuals investigated during sleep exhibited higher HRV, as indexed by the root mean square of successive differences in heart cycle duration (RMSSD) and the proportion of cycle lengths differing by more than 50 ms (pNN50) (Covassin et al., 2012). In contrast, nocturnal HRV in the high frequency band, another popular index of vagal cardiac outflow (Berntson et al., 2016), did not differ between hypotensive and normotensive samples (de Zambotti, Covassin, Cellini, Sarlo, Torre, et al., 2012). The same study documented reduced sympathovagal balance in terms of a lower ratio between the power in the low and high frequency bands of the HRV spectrum (LF/HF ratio) in hypotensives. However, the validity of the LF/HF ratio remains highly questionable (Reyes del Paso, Langewitz, Mulder, van Roon, & Duschek, 2013); as such, the findings on alterations in HRV remain equivocal and the available evidence base does not allow firm conclusions to be drawn.
The cardiac baroreflex is an additional, potentially relevant regulatory mechanism in the present context. This medullary reflex consists of a negative feedback loop, in which activity changes in arterial baroreceptors, resulting from blood pressure fluctuations, precipitate compensatory changes in heart rate and myocardial contractility (Berntson et al., 2016; Duschek, Werner, & Reyes del Paso, 2013). While the baroreflex is regarded as the most important control system in buffering short-term blood pressure fluctuations, recent research also confirmed its involvement in the setting of tonic blood pressure and the origin of essential hypertension (Carthy, 2013; Lohmeier, Irwin, Rossing, Serdar, & Kieval, 2004 ). Pilot data suggested the occurrence of increased baroreflex sensitivity (BRS) in chronic hypotension (Duschek, Dietel, Schandry, & Reyes del Paso, 2008). It has been suggested that elevated responsiveness of the reflex leads to overcompensation of phasic blood pressure increases and thus stabilization of blood pressure at a lower level. However, to date, enhanced BRS was observed only in a single study; thus, replication of this finding is certainly required.
With the goal of increasing our etiological knowledge about chronic hypotension, and taking into account the methodological limitations of the available research, the current study comprehensively quantified autonomic cardiovascular control in chronic hypotension. SV, CO, PEP, and total peripheral resistance (TPR) were obtained using impedance cardiography. While cardiac contractility is controlled by the beta-adrenergic system, TPR is mainly linked to alpha-adrenergic effects (Levy & Pappano, 2007). Even though SV is a well-established contractility index, to some extent its magnitude also depends on heart rate. Higher heart rate is related to lower ventricular preload, which, mediated by the Frank-Starling mechanism, results in lower SV (Kenny, Plappert, Doubilet, Salzman, & Sutton, 1987; Levy & Pappano, 2007). Considering this, heart rate was controlled in the statistical analysis pertaining to SV. The RMSSD derived from electrocardiography (ECG) recordings was taken as an index of HRV. This parameter represents HRV in the high frequency range and its validity as an indicator of vagal cardiac tone has been repeatedly shown (Berntson, Lozano, & Chen, 2005; Berntson et al., 2016). Finally, BRS was extrapolated from continuous blood pressure recordings using sequence analysis. This method allows estimation of BRS in the time domain based on the spontaneous covariation between systolic blood pressure and heart cycle duration (Bertinieri et al., 1985; Reyes del Paso, González, & Hernández, 2010). In order to obtain a more comprehensive picture, cardiovascular recordings were carried out under resting conditions and during
exposure to a mental challenge. This procedure also allowed for evaluation of the magnitude of cardiovascular reactivity during stress conditions, where previous research suggested blunted reactivity in chronic hypotension and thus poorer short-term autonomic cardiovascular adjustment to situational requirements (Covassin et al., 2013; Duschek, Dietel, et al., 2008; Duschek, Matthias, & Schandry, 2005). The following main hypotheses were tested in the study:
Hypothesis 1 (H1): Taking into account the research delineated above, reduced beta-adrenergic cardiac tone in chronic low blood pressure was postulated. This was expected to be expressed in lower SV and CO, and in a longer PEP in hypotensive versus normotensive individuals.
Hypothesis 2 (H2): Though the available research remains inconsistent, augmented parasympathetic influences on heart rate, indicated by higher expressions of RMSSD, were predicted in hypotension.
Hypothesis 3 (H3): Based on our pilot data, greater BRS in hypotensive versus normotensive participants was also predicted.
Hypothesis 4 (H4): Finally, reduced autonomic stress reactivity, in terms of smaller modulations in the assessed parameters during mental challenge, was postulated.
Methods
Participants
Forty subjects with hypotension, according to WHO (1978) criteria, and 40 normotensive control persons participated (35 women and 5 men in each group). None of the subjects suffered from a relevant physical disease or mental disorder. Health status was assessed by means of an anamnestic interview and a questionnaire covering diseases of the cardiovascular, respiratory, gastrointestinal, and urogenital systems, and of the thyroid and the liver, as well as metabolic diseases and psychiatric disorders. None of the participants used any kind of medication affecting the cardiovascular or central/ peripheral nervous system. In total, 67 of the participants were university students (34 in the hypotensive sample, 33 in the control group); the remaining subjects were in the workforce. Table 1 provides information about blood pressure, as recorded at the beginning of the experimental procedure, as well as age and body mass index (BMI).
Sample size was determined based on previous studies comparing parameters of autonomic control between
2017 Hogrefe Publishing Table 1 . Means (M) and standard deviations (SD) of systolic blood pressure, diastolic blood pressure, age, and body mass index in both samples
Hypotensive group Normotensive group
M SD M SD
Systolic blood pressure (mmHg) 95.41 6.69 119.18 3.82
Diastolic blood pressure (mmHg) 64.59 6.30 77.05 5.48
Age (years) 24.70 4.90 23.98 4.28 Body mass index (kg/m 2
) 20.15 1.85 21.95 2.78
hypotensive and normotensive individuals (c.f. Introduction), which revealed effect sizes (Cohen’s d) generally between .3 and .5. Assuming an effect size of .4, an α level of 5%, and a β error of 20%, power analysis revealed a required total sample size of 39 per group. The study was part of a larger project investigating psychophysiological aspects of chronic hypotension. Further results obtained in this sample are presented in Duschek, Hoffmann, and Reyes del Paso (2017) and Duschek, Hoffmann, Reyes del Paso, and Ettinger (2017).
Hemodynamic Recordings
For impedance cardiography recording, a CardioScreen 1000 (Medis Inc., Ilmenau, Germany) device was employed (c.f. Berntson et al., 2016; Moshkovitz, Kaluski, Milo, Vered, & Cotter, 2004; Raaijmakers, Faes, Scholten, Goovaerts, & Heethaar, 1999 for technical framework and discussion of method reliability and validity). The impedance signal was acquired using four spot electrodes positioned at the lateral neck and the lateral chest (left side) with alternating current of 1.5 mA and 85 kHz. The ECG was recorded at a sampling rate of1,000 Hz from two electrodes placed at the left mid-clavicle and lowest right rib using a Biopac system (MP 150, Biopac Systems Inc., Goleta, CA). The back of the left hand served as a ground. Blood pressure was monitored continuously with a Finometer Model-2 (Finapres Medical Systems, Amsterdam, The Netherlands). The cuff of the device was applied to the left index finger and that hand was positioned at the level of the heart. For periodic recalibration, the device’s “Physiocal” feature (Wesseling, De Wit, Van der Hoeven, Van Goudoever, & Settels, 1995) was in operation. Data were recorded by means of the Biopac system (sampling rate 1,000 Hz).
Procedure
screening session, which was conducted at least one week prior to the main experiment and again at the beginning of the experimental session. Here, after a rest period of 10 min, three sphygmomanometric blood pressure measurements were taken in a sitting position. For this purpose an automatic inflation blood pressure monitor (Omron M400, Omron Healthcare, Vernon Hills, IL) was used. Readings were separated by 5-min rest intervals. The mean value of the three measurements was used for group assignment. Females with a mean systolic blood pressure of less than 100 mmHg, and males with a mean value below 110 mmHg, were assigned to the hypotensive group. The inclusion criterion for the control group was systolic blood pressure between 115 and 140 mmHg. The criteria had to be fulfilled at both the screening and experimental sessions. A total of 12 prospective participants who met the inclusion criteria for the hypotensive group during screening no longer satisfied them at the experimental session. For the same reason, 17 candidates for the control group had to be excluded.
Hemodynamic recordings were accomplished at rest and during mental stress induced by a serial subtraction task. During the 7-min resting phase participants were asked to sit still, not to speak and to relax with their eyes open. The subtraction task was conducted over a 3-min period, during which subjects had to count down from 1,000, subtracting 17 each time and saying the numbers out loud. They were asked to perform the task as quickly and accurately as possible.
Experimental sessions were conducted in the morning, between 8 and 11 a.m., and in the afternoon between 2 and 5 p.m. In order to control for circadian effects, the same number of participants from both study groups was tested in the morning and afternoon. Participants were requested not to drink alcohol or beverages containing caffeine for 3 hr prior to the screening and experimental sessions. The study was approved by the Board for Ethical Questions in Science of the University of Innsbruck/Austria and all participants provided their written informed consent.
Data Analysis
The data revealed by impedance cardiography was processed using Cardio-Vascular-Lab software (Medis Inc.). SV (mL) was obtained by applying the Kubicek equation (Berntson et al., 2016), and CO (L/min) was computed by multiplying SV by heart rate. PEP (ms) was defined as the period between the onset of ventricular depolarization (Q-point in ECG) and the onset of left ventricular ejection (B-point in impedance signal). TPR (dyn s/cm 5
) was computed as (MAP/CO) 80, where MAP represents mean arterial pressure obtained by continuous finger
recording. The software provides values of the indices on a beat-to-beat basis. The data were processed further in two different ways: firstly, mean values across the resting and mental stress conditions were computed. In addition, to explore the time course of the changes in the parameters during stress induction, mean values were computed for consecutive intervals of 10 s duration each, resulting in 18 values for the mental stress period.
To aggregate the ECG data, KARDIA software (Perakakis, Joffily, Taylor, Guerra, & Vila, 2010) was employed. In a first step, values of heart cycle duration –defined as the RR interval –were computed from the raw ECG. The ECG data were visually screened, and artifacts were corrected by linear interpolation. Subsequently, values of the RMSSD (ms) were computed for the entire resting and stress conditions, and for each of the 18 consecutive 10 s intervals of the mental stress period. BRS was quantified using software developed by one of the authors (Reyes del Paso, 1994). The program locates sequences of three to six consecutive heart cycles (reflex sequences) in which systolic blood pressure increases are accompanied by increases in heart cycle duration, and those in which blood pressure decreases are accompanied by decreases in heart cycle duration. Since a time lag of one heartbeat is known to produce the best estimates of BRS (Steptoe & Vögele, 1990), each systolic value was paired with the duration of the cycle immediately following. Values of 1 mmHg and 2 ms were applied as minimal criteria for changes in blood pressure and heart cycle duration, respectively. The interval between systolic points (intersystolic interval) was taken as an index of heart cycle duration (Reyes del Paso et al., 2010). When one of these reflex sequences was detected, the regression line was computed across all heart cycles of the given sequence. BRS was expressed as the change in heart cycle duration (ms) per mmHg blood pressure change, measured by the slope of the regression line. The reliability and validity of this method has been well established (Duschek, Werner, et al., 2013; Reyes del Paso, 1994). Values of BRS (ms/mmHg) were computed for the entire resting and stress conditions and, in addition, for consecutive 30 s intervals of the stress period. Longer intervals were chosen for BRS than for the remaining parameters in order to enable reliable estimation of this parameter.
As a main instrument of statistical analysis, analysis of variance (ANOVA) models were computed with experimental group (hypotensive vs. control group) as betweensubjects factor and experimental condition (rest vs. mental stress) as the within-subjects factor. The cardiovascular indices served as dependent variables. To account for the possible dependency of SV on heart rate, an additional model was computed for SV, in which baseline heart rate was applied as a covariate. Post hoc F-tests were conducted
for all variables with significant group by condition interactions. Within both groups, values of the resting condition were compared with those of the stress period.
To describe the time course of the cardiovascular responses during stress induction, change scores were calculated as the difference between the values computed for the consecutive 10 or 30 s intervals of the stress period and corresponding mean values of the preceding rest phase. For statistical analysis, further ANOVA models were computed. Here, group served as a between-subjects factor and time (i.e., the 6 change scores of BRS and the 18 change scores of the remaining variables) as a withinsubjects factor. Alpha was set at .05 in all analyses.
Results
Figure 1 depicts the results for the indices derived from impedance cardiography, as well as heart rate (taken from the ECG recordings). SV, heart rate, and CO were lower in the hypotensive group than in the normotensive control group, whereas PEP was higher. Only a small group difference arose in TPR. While the values of heart rate and CO were higher during stress than at rest, SV and PEP decreased during the mental calculation task; TPR did not systematically change.
The ANOVA revealed a significant main effect of group for all parameters except TPR [SV: F(1, 78) = 10.22, p < .01, η 2 p = .12; heart rate: F(1, 78) = 6.88, p = .010, η 2 p = .081; CO: F(1, 78) = 47.85, p < .01, η 2 p = .38; TPR: F(1, 78) = 0.33, p = .57, η 2 p < .01; PEP: F(1, 78) = 7.69, p < .01, η 2 p = .090]. The main effect of condition was significant for all variables except TPR [SV: F(1, 78) = 18.13, p < .01, η 2 p = .19; heart rate: F(1, 78) = 89.80, p < .01, η 2 p = .54; CO: F(1, 78) = 58.45, p < .01, η 2 p = .43; TPR: F(1, 78)<.01, p = .98, η 2 p < .01; PEP: F(1, 78) = 74.42, p < .01, η 2 p = .49]. Significant group and condition effects were also observed in the additional model for SV, in which baseline heart rate was controlled for [group: F(1, 77) = 29.51, p < .01, η 2 p = .28; condition: F(1, 77) = 5.82, p = .018, η 2 p = .070]. The size of the group effect was higher than in the model in which heart rate was not controlled for. The effect of the covariate was also significant, F(1, 77) = 37.30, p < .01, η 2 p = .33. Significant group by condition effects did not arise in any of these models. In Figure 2 the values of RMSSD, BRS, and blood pressure, taken from continuous finger measurements, are displayed. While only a small group difference arose in the RMSSD, BRS was larger in hypotensive versus normotensive subjects. BRS was lower during the calculation task than at rest and systolic and diastolic blood pressure was higher. However, the extent of the blood pressure increase from rest to task was larger in the normotensive than in the hypotensive group.
The group and condition effects were significant for BRS and systolic and diastolic blood pressure, but not for the RMSSD [group: BRS, F(1, 78) = 4.99, p = .028, η 2 p = .060; RMSSD, F(1, 78) = 0.27, p = .61, η 2 p < .01; systolic blood pressure, F(1, 78) = 52.62, p < .01, η 2 p = .40; diastolic blood pressure, F(1, 78) = 21.61, p < .01, η 2 p = .22; condition: BRS, F(1, 78) = 37.83, p < .01, η 2 p = .33; RMSSD, F(1, 78) = 0.027, p = .87, η 2 p < .01; systolic blood pressure, F(1, 78) = 94.57, p < .01, η 2 p = .55; diastolic blood pressure, F(1, 78) = 126.60, p < .01, η 2 p = .62]. Among these models, significant interactions between group and condition were only found in those computed for blood pressure [systolic blood pressure: F(1, 78) = 8.48, p < .01, η 2 p = .098; diastolic blood pressure: F(1, 78) = 8.03, p < .01, η 2 p = .093]. Post hoc testing conducted separately for the hypotensive and normotensive participants revealed stress-induced increases in systolic and diastolic blood pressure in both groups; however, the effect sizes were larger in the normotensive versus hypotensive group [hypotension: systolic blood pressure, F(1) = 32.51, p < .01, η 2 p = .46; diastolic blood pressure, F(1) = 53.86, p < .01, η 2 p = .58; control group: systolic blood pressure, F(1) = 62.08, p < .01, η 2 p = .61; diastolic blood pressure, F(1) = 73.91, p < .01, η 2 p = .66]. The time courses of the changes in the cardiovascular parameters during the stress period are depicted in Figures 3 and 4. SV decreased during the first 30 s of the task period in both groups; thereafter, it progressively rose but remained below the baseline level during almost the entire period. The ANOVA revealed a main effect of time for SV, F(17, 76) = 3.09, p = .011, η 2 p = .039. 1
CO increased in both groups, peaked at the end of the first task minute, and decreased during the remaining period [time effect: F(17, 76) = 3.19, p < .01, η 2 p = .040]. PEP strongly declined in both groups, reached its minimum around 60 s after task onset, and remained at a low level toward the end of the period [time effect: F(17, 76) = 12.51, p < .01, η 2 p = .14]. TPR gradually rose beginning around 50 s after task onset; the increase was somewhat steeper in the normotensive versus hypotensive group [time effect: F(17, 76) = 17.12, p < .01, η 2 p = .18]. Both groups exhibited a steep initial heart rate increase, which reached its maximum at 20 s after task
1
The data revealed by impedance cardiography and HRV analysis from two subjects had to be excluded from the time course analysis due to missing values for certain seconds of the task interval (the noncontinuous analysis presented above was based on the available data; as such, the number of included values was somewhat reduced in these subjects). The BRS data of three subjects had to be excluded because no baroreflex sequences occurred in some of the 30 s intervals (for the analysis pertaining to the entire stress period, the number of baroreflex sequences was sufficient to reliably estimate BRS in all subjects).
100 Hypotension Control group
90
Stroke volume (ml)
80
70
60
50
Rest Mental stress
Cardiac output (l/min)
1 2 3 4 5 6 7 8
Rest Mental stress
1100
1000
Total peripheral resistance (dyn x s/cm 5 )
900
800
700
600
Rest Mental stress 140
130
Pre-ejection period (ms)
120
110
100
Rest Mental stress
60 65 70 75 80 85 90 95
Heart rate (beats/min)
Rest Mental stress
Figure 1. Mean values of stroke volume, cardiac output, total peripheral resistance, pre-ejection period, and heart rate (bars denote standard errors of the mean).
onset. Between 40 s and 100 s, heart rate decreased once again, and remained stable until the end of the period [time effect: F(17, 78) = 20.60, p < .01, η 2 p = .21]. While BRS progressively declined in both groups during the entire task, [time effect: F(5, 75) = 6.87, p < .01, η 2 p = .084], RMSSD decreased during the initial task phase and thereafter gradually increased [time effect: F(17, 76) = 6.40, p < .01, η 2 p = .078]. Systolic and diastolic blood pressure progressively rose during the task in both groups [time effect systolic blood pressure: F(17, 78) = 47.26, p < .01, η 2 p = .38; time effect diastolic blood pressure: F(17, 78) = 44.28, p < .01, η 2 p = .36]. However, the slope of the increase was markedly higher in the control group versus the hypotensive group, as confirmed by significant group and interaction effects [group effect of systolic blood pressure: F(1, 78) = 8.31,
p < .01, η 2 p = .096; group effect of diastolic blood pressure: F(1, 78) = 6.24, p = .015, η 2 p = .074; interaction effect of systolic blood pressure: F(17, 78) = 4.51, p < .01, η 2 p = .055; and interaction effect of diastolic blood pressure: F(17, 78) = 3.30, p < .04, η 2 p = .041]. No group or interaction effects were obtained for the remaining variables.
Discussion
With the aim of extending our knowledge regarding the relevance of autonomic dysregulation to chronic hypotension, this study compared parameters of sympathetic and parasympathetic cardiovascular control and baroreflex
7 9 11 13 15 17 19
Rest Mental stress Baroreflex senstitivity (ms/mmHg) Hypotension Control group
RMSSD (ms)
0 10 20 30 40 50 60
Rest Mental stress
Systolic blood pressure (mmHg)
70 80 90 100 110 120 130
Rest Mental stress
Diastolic blood pressure (mmHg)
80
70
60
50
40
30
Rest Mental stress
Figure 2. Mean values of baroreflex sensitivity, root mean square of successive differences in heart cycle duration (RMSSD), systolic and diastolic blood pressure (bars denote standard errors of the mean).
function between individuals with blood pressure in the hypotensive and normotensive ranges. While hypotension was associated with markedly lower SV, heart rate, and CO, as well as with higher PEP and BRS, no group differences arose in TPR and high frequency HRV indexed by the RMSSD. According to these results, reduced betaadrenergic myocardial drive and increased baroreflex function stand to reason as being the principal components of the autonomic impairment associated with chronic hypotension.
The study confirmed previous findings of reductions in SV and CO in chronic hypotension at rest and during mental stress (Duschek, Heiss, et al., 2009). These findings, however, had to be regarded as preliminary, given that SV was derived from blood pressure waveforms using Modelflow analysis (Wesseling, Jansen, Settels, & Schreuder, 1993). While this method allows relatively precise assessment of intra-individual hemodynamic changes, it provides only a rough estimation of absolute values; as such, its suitability for the assessment of interindividual differences in hemodynamic function is confined to tight limits (Bogert & Lieshout, 2005). For the latter purpose, the impedance cardiography presently applied is clearly more appropriate (Moshkovitz et al., 2004). This method provides reliable hemodynamic measures, which are highly correlated with those of echocardiography or invasive techniques
(Berntson et al., 2016; Moshkovitz et al., 2004; Raaijmakers et al., 1999). Even though SV varies subject to myocardial contractility, which underlies beta-adrenergic control, to some degree it is also influenced by heart rate (Berntson et al., 2016; Hassan & Turner, 1983). Therefore, heart rate was statistically controlled for in the present analysis, which led to an even larger effect size in the group comparison. PEP is inversely related to beta-adrenergic activity (Levy & Pappano, 2007); as such, its presently observed reduction also reflects lower sympathetic cardiac drive in hypotension.
The diminished SV together with the lower heart rate caused a reduction in CO by 20% at rest and 18% during mental challenge in the hypotensive versus control group. It has been argued that diminished organ perfusion due to lower CO accounts for some of the complaints that typically accompany chronically low blood pressure (Covassin et al., 2012; Duschek, Heiss, et al., 2009). This may be the case, for example, for low skin temperatures and the experience of cold limbs. Reduced cerebral blood flow in hypotension may be another consequence (Duschek & Schandry, 2004, 2007). Cerebral hypo-perfusion may also be relevant in terms of the association between hypotension and geriatric cognitive disorders, which has repeatedly been reported (Heijer et al., 2003; Jochemsen et al., 2013; Muller et al., 2014; Qiu, Winblad, & Fratiglioni, 2005).
Figure 3. Time course of the changes in stroke volume, cardiac output, total peripheral resistance, pre-ejection period, and heart rate during mental stress (absolute changes with respect to baseline; bars denote standard errors of the mean).
However, it should be noted that these studies were based on samples that did not represent the population currently addressed. In most samples in research on late-life hypotension, this state was preceded by mid-life cardiovascular and cerebrovascular disease or hypertension. These conditions may cause brain atrophy and cognitive decline in older age, for example, due to vascular changes or
impaired cerebral autoregulation. As such, it has to be considered that behavioral and physiological alterations associated with geriatric hypotension are mediated by mechanisms different from those occurring in young individuals with chronic hypotension.
Confirming our previous observation (Duschek, Heiss, et al., 2009), the current study groups did not differ in
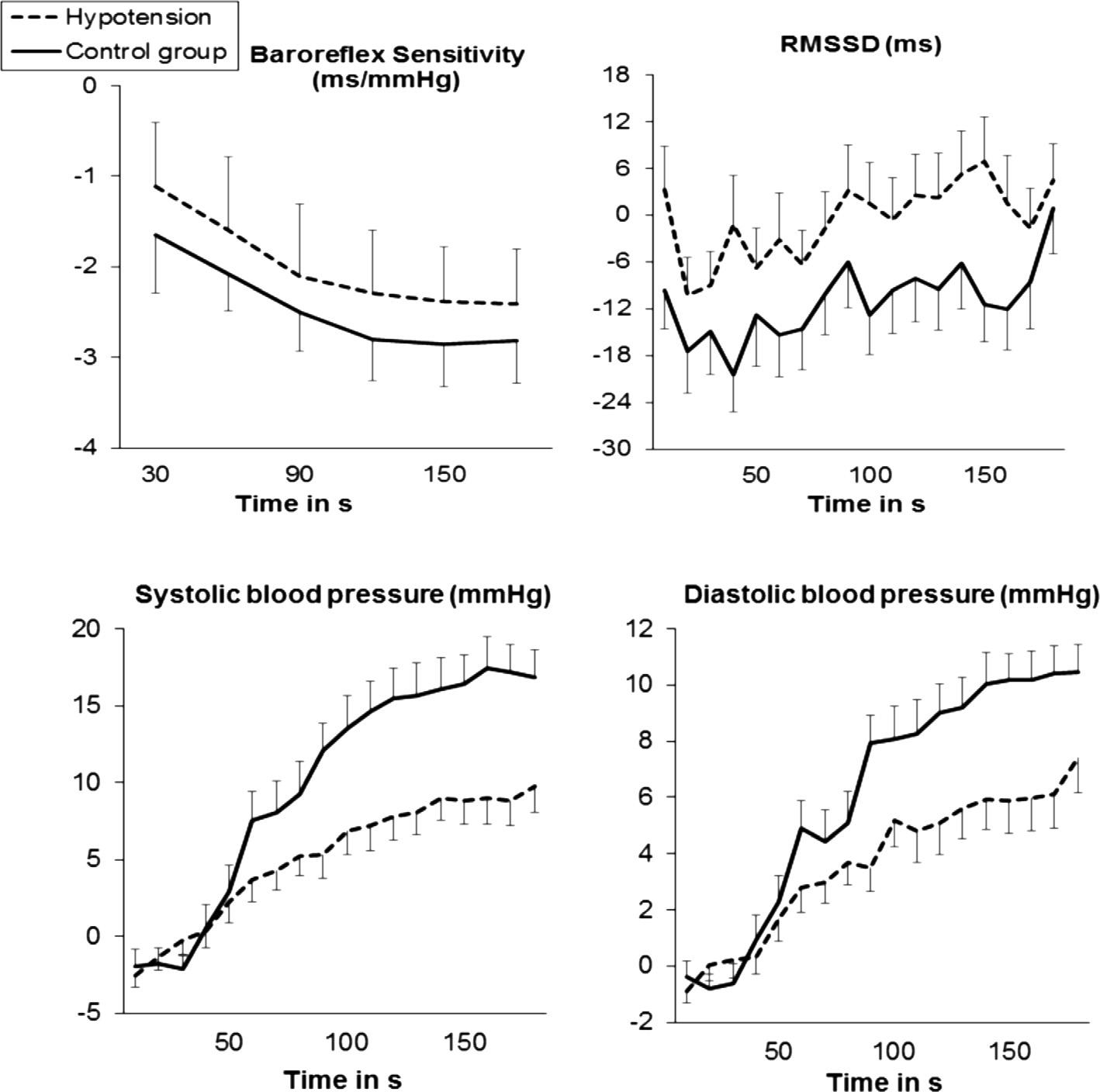
Figure 4. Time course of the changes in baroreflex sensitivity, root mean square of successive differences in heart cycle duration (RMSSD), systolic and diastolic blood pressure during mental stress (absolute changes with respect to baseline; bars denote standard errors of the mean).
TPR. The pattern of reduced SV and CO and normal TPR has been considered to be indicative of the hemodynamic origin of chronic hypotension (Covassin et al., 2012; Duschek, Heiss, et al., 2009). Accordingly, this state may mainly be caused by cardiac factors, whereas vascular regulation may only play a subordinate role. This hypothesis has been partially challenged by the study of Covassin et al. (2012) on nocturnal autonomic cardiovascular control. The comparison between hypotensive and normotensive individuals did not reveal an overall difference in TPR recorded during 7 hr of sleep; however, groups differed in the temporal profile of TPR changes. In contrast to controls, hypotensive participants demonstrated progressive rise in TPR especially during the second half of the night, which was interpreted as a compensatory response to the drop in CO which occurred over time. On this account, aberrances in the regulation of vascular tone mediated by the alpha-adrenergic system in hypotension cannot be completely ruled out.
Research on autonomic dysregulation in prehypertension may also be of interest in the present context.
Prehypertension has been defined by systolic blood pressure between 120 and 139 mmHg and diastolic blood pressure between 80 and 89 mmHg (Chobanian et al., 2003). It has been implicated in increased risk of cardiovascular disease (Qureshi, Suri, Kirmani, & Divani, 2005; Vasan, Larson, Leip, Kannel, & Levy, 2001), which is supported by meta-analytic evidence showing that the linear relationship between blood pressure and vascular mortality not only exists in hypertension, but also in the prehypertensive range (Prospective Studies Collaboration, 2002). It has been argued that sympathetic cardiac control is involved in the genesis of this state. Observations of increased norepinephrine levels, in addition to elevated CO due to enhanced cardiac contractility and higher heart rate, confirmed this notion (Davis et al., 2012; Drukteinis et al., 2007). It has been shown that prehypertension tends to rapidly progress to chronic hypertension (Vasan et al., 2001). This is consistent with the view that sympathetically mediated augmentation of CO contributes to hypertension during its early phase (Lovallo & al’Absi, 1998; Lund-Johansen, 1991). Available findings on TPR in
prehypertension are still inconsistent (Davis et al., 2012; Drukteinis et al., 2007), whereas it has been well established that augmented TPR constitutes the most relevant hemodynamic aberrance in chronically elevated blood pressure, at least during the chronic phase (Cain & Khalil, 2002). Regarding the present study, it may be noteworthy that the inclusion criteria of the control group also encompassed the prehypertensive range. Therefore, a question arises as to the degree to which group differences in cardiac sympathetic tone and blood pressure reactivity were driven by controls expressing signs of autonomic dysregulation in prehypertension. The blood pressure of seven (17.5%) controls fell in the prehypertensive range at the beginning of the experimental session; only three (7.5%) of them fulfilled these criteria at both the screening and experimental sessions (Chobanian et al., 2003). Considering these relatively low numbers, one may conclude that the impact of prehypertension on the group effects was rather limited and that they arose mainly due to autonomic differences between hypotensive and normotensive participants.
Replicating a former finding (Duschek, Dietel, et al., 2008), BRS was markedly higher in the present hypotensive sample. In addition to the aforementioned blood pressure reduction due to overcompensation of phasic blood pressure increases (in the case of enhanced BRS), the possibility that the operating point of the baroreflex is reset to lower blood pressure values has been considered to contribute to hypotension development (Duschek, Dietel, et al., 2008). The relevance of the baroreflex system to blood pressure over the long term is emphasized by the wellknown reflex inhibition that occurs in essential hypertension (Carthy, 2013), as well as the inverse relationship between BRS and tonic blood pressure in healthy normotensive individuals (Duschek & Reyes del Paso, 2007; Hesse, Charkoudian, Liu, Joyner, & Eisenach, 2007). In a follow-up study, low BRS predicted blood pressure increases over a 5-year period (Ducher, Fauvel, & Cerrutti, 2006). Finally, experimental observations in animals indicated that prolonged stimulation of the baroreceptor afferents leads to sustained blood pressure decline (Lohmeier et al., 2004). In addition to its validity as a psychophysiological indicator of health and disease, BRS has a proven impact on various features of behavior regulation (Duschek, Werner, et al., 2013). In the present context, for example, the repeatedly documented inverse association between BRS and cognitive performance may be of interest (Duschek, Muckenthaler, & Reyes del Paso, 2009; Reyes del Paso, González, Hernández, Duschek, & Gutiérrez, 2009; Yasumasu, Reyes del Paso, Takahara, & Nakashima, 2006). This connection has been discussed in the context of the well-established central nervous inhibition that arises from baroreceptor afferents, where more pronounced
inhibition seems to occur in the case of higher BRS (Duschek, Werner, et al., 2013). This mechanism may also play a role in hypotension-related cognitive impairment (Duschek et al., 2005). Similarly, interindividual differences in cortical activation processes, as assessed by evoked EEG potentials, correlated negatively with BRS (Duschek, Wörsching, & Reyes del Paso, 2013). Baroreceptormediated inhibition may also account for this association; as such, the enhanced BRS in hypotension may be involved in the reduced cortical activation observed in this population (Duschek et al., 2006; Weisz, Schandry, Jacobs, Mialet, & Duschek, 2002). In contrast to BRS, RMSSD did not differ between the two study groups. Although the RMSSD represents overall HRV, it is most closely associated with HRV in the frequency range of respiration, and constitutes a wellestablished index of parasympathetic influences on heart rate (Berntson et al., 2016). Previous analyses of HRV in chronic hypotension yielded inconsistent results. While ECG recordings during sleep revealed higher values of RMSSD and pNN50, indicating enhanced cardiac vagal outflow to the sinus node (Covassin et al., 2012), no effects were found for nocturnal high frequency HRV (de Zambotti, Covassin, Cellini, Sarlo, Torre, et al., 2012). The present observations, of no group difference in high frequency HRV but increased BRS in hypotension, may seem counterintuitive, as these parameters are closely linked physiologically (Reyes del Paso, Hernández, & González, 2004; Reyes del Paso, Langewitz, Robles, & Perez, 1996). BRS and RSA commonly show substantial positive correlations, increasing and decreasing in parallel under conditions of mental challenge, relaxation, or pharmacological treatment (Duschek, Heiss, et al., 2009; Reyes del Paso et al., 1996, 2004). The baroreflex is believed to be one of the most important sources of HRV in the frequency range of respiration (Berntson et al., 2016; Reyes del Paso et al., 2013; van Roon, Mulder, Althaus, & Mulder, 2004). Given its rapid response characteristics, the reflex is able to phasically alter cardiac vagal outflow even at the highest frequencies. Baroreflex activity is modulated by respiratory influences of both central nervous and peripheral origin, which trigger cyclical fluctuations in the discharge rates of vagal motoneurons (Berntson et al., 1997). Despite these causal influences of the baroreflex on HRV, dissociations between BRS and high frequency HRV have been repeatedly reported. For example, it was observed that BRS and high frequency HRV correlated in opposite directions with EEG measures of cortical tone and cortical excitability (Duschek, Wörsching, et al., 2013; Duschek, Wörsching, & Reyes del Paso, 2015). Furthermore, specific associations of both parameters with cognitive performance and emotional states have been demonstrated (Delgado, Vila, & Reyes del Paso, 2014;
Duschek, Muckenthaler, & Reyes del Paso, 2009; Yasumasu et al., 2006). It is therefore evident that high frequency HRV does not exclusively reflect the properties of the baroreflex; BRS and HRV affect psychological and physiological processes via different pathways (Duschek, Wörsching, et al., 2013; Duschek et al., 2015). Accordingly, both parameters at least partly represent different mechanisms of cardiac regulation, where baroreflex mechanisms seem to play a more prominent role in the genesis of hypotension than processes represented by HRV.
In the present study various changes in cardiovascular parameters during mental stress were observed. The higher CO and lower PEP during stress versus baseline denote stress-induced sympathetic activation (Berntson et al., 2016). RMSSD did not differ between baseline and stress conditions. Though one would expect vagal withdrawal during mental effort, a similar finding was reported by de Zambotti, Covassin, Cellini, Sarlo, and Stegagno (2012). In hypotensive and normotensive women, HRV in the high frequency range did not significantly change between rest and cognitive stress induced by a 2-back bask. High frequency HRV is a well-established index of vagal influences on heart rate that correlates highly with the RMSSD (Berntson et al., 2005, 2016). While de Zambotti, Covassin, Cellini, Sarlo, and Stegagno (2012) also observed stress-induced heart rate modulation comparable to the present study, PEP was largely unaffected. BRS was lower during the stress than resting conditions; this is commonly seen under conditions of mental activity and other laboratory stressors (Brooks, Fox, Lopez, & Sleight, 1978; Duschek & Reyes del Paso, 2007; Robbe et al., 1987). BRS attrition implies a reduced buffering effect of the baroreflex, which facilitates stress-induced heart rate and blood pressure increases, and by extension enhancement of the metabolic supply of the organism, thereby also improving conditions for neural and cognitive processing (Duschek, Werner, et al., 2013). Interestingly, in both study groups, SV was lower during stress than at rest. This may be explained by the fact that heart rate acceleration is associated with a reduction in ventricular preload, which, due to the FrankStarling mechanism, leads to lower SV (Kenny et al., 1987; Levy & Pappano, 2007). The extent of the increase in systolic and diastolic blood pressure during cognitive challenge was smaller in hypotensive versus control subjects. This is consistent with previous reports of blunted cardiovascular reactivity in chronic hypotension, which has been interpreted in terms of insufficient hemodynamic adjustment to current requirements (Covassin et al., 2013; Duschek, Dietel, et al., 2008; Duschek et al., 2005). Reduced blood pressure reactivity may also be involved in deficient cerebral blood flow regulation in hypotension. Various studies using transcranial Doppler sonography have documented blunted hemodynamic
adjustment, in terms of smaller phasic blood flow increases during cognitive and emotional processing (Cellini et al., 2015; Duschek & Schandry, 2004; Sarlo, de Zambotti, Gallicchio, Devigili, & Stegagno, 2013). Moreover, recent research indicated that the amplitudes of cerebral blood flow responses depend, to a certain degree, on the magnitude of blood pressure reactions (Duschek, Heiss, Schmidt, Werner, & Schuepbach, 2010; Duschek & Schandry, 2006; Duschek, Werner, Kapan, & Reyes del Paso, 2008). This is relevant insofar as it has repeatedly been shown that performance related to attentional and executive functions varies according to the magnitude of cerebral blood flow responses, where insufficient blood flow adjustment plays an important role in hypotension-related cognitive impairment (Duschek, Hadjamu, & Schandry, 2007 a; Duschek & Schandry, 2004; Duschek, Schuepbach, & Schandry, 2008; Schuepbach, Boeker, Duschek, & Hell, 2007). The analysis of the time courses of the cardiovascular indices during mental stress revealed various patterns of changes. Heart rate and CO steeply increased during the initial phase of the arithmetic task, and thereafter decreased before reaching a plateau slightly above baseline. In contrast, RMSSD markedly decreased during the initial phase, and gradually rose during the remaining task. PEP also showed a strong initial decline but remained at a low level until the end of the period. Other variables displayed slower changes: BRS progressively decreased during the entire task, whereas TPR –as well as systolic and diastolic blood pressure –gradually increased. The smaller slope of the blood pressure rise in the hypotensive versus normotensive sample confirms the notion of blunted blood pressure reactivity in this condition (Duschek et al., 2005). The different time dynamics reflect contributions of different physiological mechanisms to the cardiovascular modulations. The rapid changes immediately after task onset observed in heart rate, SV, and PEP may have been evoked by fastacting regulatory mechanisms, especially the autonomic nervous system. Specifically, such changes may occur due to alpha- and beta-sympathetic activation, as well as vagal withdrawal in the case of heart rate. Progressive reduction of vagal inhibition after the initial task period may have contributed to heart rate recovery. This is supported by the modulation of RMSSD, characterized by a steep initial decline and progressive increase across the remaining period. The gradual increases in TPR and blood pressure during the task reflect the involvement of slower mechanisms, such as activation of adrenal medullary hormones (Berntson et al., 2016). Reduction in BRS during mental challenge is commonly ascribed to top-down modulation of cardiovascular brain stem centers by cortical units (Duschek, Werner, & Reyes del Paso, 2013; van Roon et al., 2004). Progressive BRS decrease implies a reduction in the compensatory effect of the baroreflex, which allows
blood pressure to increase. This mechanism is illustrated by the inverse time courses of BRS and blood pressure across the task period.
Some methodological limitations of the study warrant discussion. The WHO (1978) criteria, which were applied during recruitment of hypotensive individuals, that is, systolic blood pressure below 100 mmHg in women and 110 mmHg in men, are relatively lenient (Covassin et al., 2012, 2013). More recently proposed definitions include lower systolic cut-off values and also take into account the diastolic value. For example, the US National Heart, Lung, and Blood Institute (NHLBI) suggested 90 and 60 mmHg as systolic and diastolic limits (NHLBI, 2017). The application of more strict criteria would potentially reveal a different pattern of autonomic dysregulation, and the presently investigated sample is not representative of hypotensives defined according to stricter criteria, such as those of the NHLBI (2017). Another limitation pertains to the lack of assessment of subjective hypotensive complaints; therefore, it was not possible to establish the impact of autonomic alterations on symptom severity. Regarding cardiovascular recordings, it must be acknowledged that blood volume was not measured, which impeded the investigation of its possible role in mediating group differences in hemodynamic parameters. Furthermore, respiration rate was not controlled for during computation of the RMSSD. Even though recent research did not support the utility of respiration control in HRV assessment (Denver, Reed, & Porges, 2007), possible distortion of the findings, due to respiratory changes occurring during the verbal response required in the serial subtraction task, cannot be ruled out.
In short, the present study identified reduced sympathetic myocardial drive as the main characteristic of the autonomic impairment associated with chronic hypotension, where diminished beta-adrenergic inotropic influence, rather than aberrant alpha-adrenergic vasomotor control, may play a dominant role. While the study groups did not differ in HRV, involvement of the parasympathetic system in this condition is suggested by increased baroreflex function. Finally, the possible implications for the pharmacological treatment of chronic hypotension should be considered; the data indicate greater suitability of beta- versus alpha sympathomimetic agents. This is supported by pilot data on the acute effects of different sympathomimetics in chronic hypotension. While beta-agonistic treatment was followed by heart rate and blood pressure elevation (Duschek, Hadjamu, & Schandry, 2007b), acute application of an alpha-agonist led to extreme baroreflex-mediated heart rate deceleration, which in turn impeded enhancement of CO (Duschek, Heiss, et al., 2009). However, as chronic hypotension does not constitute a serious medical condition, the necessity for sympathomimetic treatment is certainly restricted to a very limited number of cases.
Furthermore, empirical knowledge on potential benefits for subjective complaints, as well as possible undesirable effects, remains scant (Duschek & Schandry, 2007; Duschek, Heiss, et al., 2009); as such, further research is clearly warranted.
Acknowledgments The study was supported by the Anniversary Fund of the Austrian National Bank (project 16289). We are grateful to Maximilian Stefani and Angela Bair for their help with data analysis.
Ethics and Disclosure Statements All participants of the study provided written informed consent prior to the study and the study was approved by the Board for Ethical Questions in Science of the University of Innsbruck (Austria).
All authors disclose no actual or potential conflicts of interest including any financial, personal, or other relationships with other people or organizations that could inappropriately influence (bias) their work.
References
Berntson, G. G., Bigger, J. T. Jr., Eckberg, D. L., Grossman, P., Kaufmann, P. G., Malik, M., ... van der Molen, M. W. (1997). Heart rate variability: Origins, methods, and interpretive caveats. Psychophysiology, 34, 623–648. Berntson, G. G., Lozano, D. L., & Chen, Y. J. (2005). Filter properties of root mean square successive difference (RMSSD) for heart rate. Psychophysiology, 42, 246–252. https://doi.org/ 10.1111/j.1469-8986.2005.00277.x Berntson, G. G., Quigley, K. S., Norman, G. J., & Lozano, D. (2016). Cardiovascular psychophysiology. In J. T. Cacioppo, L. G. Tassinary, & G. G. Berntson (Eds.), Handbook of psychophysiology (pp. 183–215). Cambridge, UK: Cambridge University Press. Bertinieri, G., di Rienzo, M., Cavallazzi, A., Ferrari, A. U., Pedotti, A., & Mancia, G. (1985). A new approach to analysis of the arterial baroreflex. Journal of Hypertension, 3, S79–S81. Bogert, L. W. J., & Lieshout, J. J. (2005). Non-invasive arterial pressure and stroke volume changes from the human finger. Experimental Physiology, 90, 437–446. https://doi.org/ 10.1113/expphysiol.2005.030262 Brooks, D., Fox, P., Lopez, R., & Sleight, P. (1978). The effect of mental arithmetic on blood pressure variability and baroreflex sensitivity in man. Journal of Physiology, 280, 75–76. Cacioppo, J. T., Berntson, G. G., Binkley, P. F., Quigley, K. S., Uchino, B. N., & Fieldstone, A. (1994). Autonomic cardiac control. II. Noninvasive indices and basal response as revealed by autonomic blockades. Psychophysiology, 31 , 586–598. https://doi.org/10.1111/j.1469-8986.1994.tb02351.x Cain, A. E., & Khalil, R. A. (2002). Pathophysiology of essential hypertension: Role of the pump, the vessel, and the kidney. Seminars in Nephrology, 22, 3–16. Carthy, E. R. (2013). Autonomic dysfunction in essential hypertension: A systematic review. Annals of Medicine & Surgery, 3, 2–7. https://doi.org/10.1016/j.amsu.2013.11.002 Cellini, N., de Zambotti, M., Covassin, N., Gallicchio, G., Stegagno, L., & Sarlo, M. (2015). Reduced cerebral and cardiovascular
hemodynamics during sustained affective stimulation in young women with chronic low blood pressure. Physiology & Behavior, 143, 83–89. https://doi.org/10.1016/j.physbeh.2015. 02.047 Chobanian, A. V., Bakris, G. L., Black, H. R., Cushman, W. C., Green, L. A., Izzo, J. L., ... Roccella, E. J. (2003). Seventh report of the Joint National Committee on Prevention, Detection, Evaluation, and Treatment of High Blood Pressure. Hypertension, 42, 1206–1252. https://doi.org/10.1161/01.HYP.0000107251. 49515.c2 Covassin, N., de Zambotti, M., Cellini, N., Sarlo, M., & Stegagno, L. (2012). Nocturnal cardiovascular activity in essential hypotension: Evidence of differential autonomic regulation. Psychosomatic Medicine, 74, 952–960. https://doi.org/10.1097/PSY. 0b013e318272db69 Covassin, N., de Zambotti, M., Cellini, N., Sarlo, M., & Stegagno, L. (2013). Cardiovascular down-regulation in essential hypotension: Relationships with autonomic control and sleep. Psychophysiology, 50, 767–776. https://doi.org/10.1111/ psyp.12055 Davis, J. T., Rao, F., Naqshbandi, D., Fung, M. M., Zhang, K., Schork, A. J., ... O’Connor, D. T. (2012). Autonomic and hemodynamic origins of pre-hypertension: Central role of heredity. Journal of the American College of Cardiology, 59, 2206–2216. https://doi.org/10.1016/j.jacc.2012.02.040 De Buyzere, M., Clement, D. L., & Duprez, D. (1998). Chronic low blood pressure: A review. Cardiovascular Drugs and Therapy, 12, 29–35. https://doi.org/10.1023/A:1007729229483 Delgado, L. C., Vila, J., & Reyes del Paso, G. A. (2014). Proneness to worry is negatively associated with blood pressure and baroreflex sensitivity: Further evidence of the blood pressure emotional dampening hypothesis. Biological Psychology, 96, 20–27. https://doi.org/10.1016/j.biopsycho.2013.11.005 Denver, J. W., Reed, S. F., & Porges, S. W. (2007). Methodological issues in the quantification of respiratory sinus arrhythmia. Biological Psychology, 74, 286–294. https://doi.org/10.1016/ j.biopsycho.2005.09.005 de Zambotti, M., Covassin, N., Cellini, N., Sarlo, M., & Stegagno, L. (2012). Cardiac autonomic profile during rest and working memory load in essential hypotensive women. International Journal of Psychophysiology, 85, 200–205. https://doi.org/ 10.1016/j.ijpsycho.2012.05.002 de Zambotti, M., Covassin, N., Cellini, N., Sarlo, M., Torre, J., & Stegagno, L. (2012). Hemodynamic and autonomic modifications during sleep stages in young hypotensive women. Biological Psychology, 91 , 22–27. https://doi.org/10.1016/ j.biopsycho.2012.05.009 Drukteinis, J. S., Roman, M. J., Fabsitz, R. R., Lee, E. T., Best, L. G., Russell, M., & Devereux, R. B. (2007). Cardiac and systemic hemodynamic characteristics of hypertension and prehypertension in adolescents and young adults: The Strong Heart Study. Circulation, 115, 221 –227. https://doi.org/ 10.1161/CIRCULATIONAHA.106.668921 Ducher, M., Fauvel, J. P., & Cerrutti, C. (2006). Risk profile in hypertension genesis: A five-year follow-up study. American Journal of Hypertension, 19, 775–781. https://doi.org/10.1016/ j.amjhyper.2005.07.019 Duschek, S., Dietel, A., Schandry, R., & Reyes del Paso, G. A. (2008). Increased baroreflex sensitivity and reduced cardiovascular reactivity in chronic low blood pressure. Hypertension Research, 31 , 1873–1878. https://doi.org/10.1291/hypres.31.1873 Duschek, S., Hadjamu, M., & Schandry, R. (2007a). Enhancement of cerebral blood flow and cognitive performance due to pharmacological blood pressure elevation in chronic hypotension. Psychophysiology, 44, 145–153. https://doi.org/10.1111/ j.1469-8986.2006.00472.x
2017 Hogrefe Publishing Duschek, S., Hadjamu, M., & Schandry, R. (2007b). Dissociation between cortical activation and cognitive performance in the pharmacological treatment of chronic hypotension. Biological Psychology, 75, 277–285. https://doi.org/10.1016/j.biopsycho. 2007.03.007 Duschek, S., Heiss, H., Buechner, B., Werner, N. S., Schandry, R., & Reyes del Paso, G. A. (2009). Hemodynamic determinants of chronic hypotension and their modification through vasopressor application. Journal of Physiological Sciences, 59, 105–112. https://doi.org/10.1007/s12576-008-0015-5 Duschek, S., Heiss, H., Schmidt, F. H., Werner, N., & Schuepbach, D. (2010). Interactions between systemic hemodynamics and cerebral blood flow during attentional processing. Psychophysiology, 47, 1159–1166. https://doi.org/10.1111/j.1469-8986. 2010.01020.x Duschek, S., Hoffmann, A., & Reyes del Paso, G. A. (2017). Affective impairment in chronically low blood pressure. Journal of Psychosomatic Research, 93, 33–40. https://doi.org/ 10.1016/j.jpsychores.2016.12.008 Duschek, S., Hoffmann, A., Reyes del Paso, G. A., & Ettinger, U. (2017). Autonomic cardiovascular control and executive function in chronically low blood pressure. Annals of Behavioral Medicine, 51 , 442–453. Duschek, S., Matthias, E., & Schandry, R. (2005). Essential hypotension is accompanied by deficits in attention and working memory. Behavioral Medicine, 30, 149–158. https:// doi.org/10.3200/BMED.30.4.149-160 Duschek, S., Meinhardt, J., & Schandry, R. (2006). Reduced cortical activity due to chronic low blood pressure: An EEG study. Biological Psychology, 72, 241 –250. https://doi.org/ 10.1016/j.biopsycho.2005.06.011 Duschek, S., Muckenthaler, M., & Reyes del Paso, G. A. (2009). Relationships between features of cardiovascular control and cognitive performance. Biological Psychology, 81 , 110–117. https://doi.org/10.1016/j.biopsycho.2009.03.003 Duschek, S., & Reyes del Paso, G. A. (2007). Quantification of cardiac baroreflex function at rest and during autonomic stimulation. Journal of Physiological Sciences, 57, 259–268. https://doi.org/10.2170/physiolsci.RP008807 Duschek, S., & Schandry, R. (2004). Cognitive performance and cerebral blood flow in essential hypotension. Psychophysiology, 41 , 905–913. https://doi.org/10.1111/j.1469-8986.2004. 00249.x Duschek, S., & Schandry, R. (2006). Deficient adjustment of cerebral blood flow to cognitive activity due to chronically low blood pressure. Biological Psychology, 72, 311 –317. https://doi. org/10.1016/j.biopsycho.2005.12.003 Duschek, S., & Schandry, R. (2007). Reduced brain perfusion and cognitive performance due to essential hypotension. Clinical Autonomic Research, 17, 69–76. https://doi.org/10.1007/ s10286-006-0379-7 Duschek, S., Schuepbach, D., & Schandry, R. (2008). Time-locked association between rapid cerebral blood flow modulation and attentional performance. Clinical Neurophysiology, 119, 1292–1299. https://doi.org/10.1016/j.clinph.2008.01.102 Duschek, S., Weisz, N., & Schandry, R. (2003). Reduced cognitive performance and prolonged reaction time accompany moderate hypotension. Clinical Autonomic Research, 13, 427–432. https://doi.org/10.1007/s10286-003-0124-4 Duschek, S., Werner, N., Kapan, N., & Reyes del Paso, G. A. (2008). Patterns of cerebral blood flow and systemic hemodynamics during arithmetic processing. Journal of Psychophysiology, 22, 81 –90. https://doi.org/10.1027/0269-8803.22.2.81 Duschek, S., Werner, N. S., & Reyes del Paso, G. A. (2013). The behavioral impact of baroreflex function: A review. Psychophysiology, 50, 1183–1193. https://doi.org/10.1111/psyp.12136
Duschek, S., Wörsching, J., & Reyes del Paso, G. A. (2013). Interactions between autonomic cardiovascular regulation and cortical activity: a CNV study. Psychophysiology, 50, 388–397. https://doi.org/10.1111/psyp.12026 Duschek, S., Wörsching, J., & Reyes del Paso, G. A. (2015). Autonomic cardiovascular regulation and cortical tone. Clinical Physiology and Functional Imaging, 35, 383–392. https://doi. org/10.1111/cpf.12174 Freeman, R., Wieling, W., Axelrod, F. B., Benditt, D. G., Benarroch, E., Biaggioni, I., ... van Dijk, J. G. (2011). Consensus statement on the definition of orthostatic hypotension, neurally mediated syncope and the postural tachycardia syndrome. Clinical Autonomic Research, 21 , 69–72. Hassan, S., & Turner, P. (1983). Systolic time intervals: A review of the method in the non-invasive investigation of cardiac function in health, disease and clinical pharmacology. Postgraduate Medical Journal, 59, 423–434. Heijer, T., Skoog, I., Oudkerk, M., de Leeuw, F. E., de Groot, J. C., Hofman, A., & Breteler, M. M. (2003). Association between blood pressure levels over time and brain atrophy in the elderly. Neurobiology of Aging, 24, 307–313. https://doi.org/10.1016/ S0197-4580(02)00088-X Hesse, C., Charkoudian, N., Liu, Z., Joyner, M. J., & Eisenach, J. H. (2007). Baroreflex sensitivity inversely correlates with ambulatory blood pressure in healthy normotensive humans. Hypertension, 50, 41 –46. https://doi.org/10.1161/HYPERTENSIONAHA.107. 090308 Jochemsen, H. M., Muller, M., Visseren, F. L., Scheltens, P., Vincken, K. L., Mali, W. P., ... Geerlings, M. I. (2013). Blood pressure and progression of brain atrophy: The SMART-MR Study. JAMA Neurology, 70, 1046–1053. https://doi.org/ 10.1001/jamaneurol.2013.217 Kenny, J., Plappert, T., Doubilet, P., Salzman, D., & Sutton, M. (1987). Effects of heart rate on ventricular size, stroke volume, and output in the normal human fetus: A prospective Doppler echocardiographic study. Circulation, 76, 52–58. https://doi. org/10.1161/01.CIR.76.1.52 Levy, M. N., & Pappano, A. J. (2007). Cardiovascular physiology. Philadelphia, PA: Mosby Elsevier. Lohmeier, T. E., Irwin, E. D., Rossing, M. A., Serdar, D. J., & Kieval, R. S. (2004). Prolonged activation of the baroreflex produces sustained hypotension. Hypertension, 43, 306–311. https://doi. org/10.1161/01.HYP.0000111837.73693.9b Lovallo, W. R., & al’Absi, M. (1998). Hemodynamics during rest and behavioral stress in normotensive men at high risk for hypertension. Psychophysiology, 35, 47–53. Lund-Johansen, P. (1991). Twenty-year follow-up of hemodynamics in essential hypertension during rest and exercise. Hypertension, 18, SIII54–SIII61. Moshkovitz, Y., Kaluski, E., Milo, O., Vered, Z., & Cotter, G. (2004). Recent developments in cardiac output determination by bioimpedance: Comparison with invasive cardiac output and potential cardiovascular applications. Current Opinion in Cardiology, 19 , 229–237. https://doi.org/10.1097/00001573- 200405000-00008 Muller, M., Sigurdsson, S., Kjartansson, O., Aspelund, T., Lopez, O. L., Jonnson, P. V., ... Launer, L. J. (2014). Joint effect of midand late-life blood pressure on the brain: The AGES-Reykjavik study. Neurology, 82, 2187–2195. https://doi.org/10.1212/ WNL.0000000000000517 National Heart, Lung, and Blood Institute. (2017). What is hypotension? Retrieved from https://www.nhlbi.nih.gov/ health/health-topics/topics/hyp Pemberton, J. (1989). Does constitutional hypotension exist? British Medical Journal, 298, 660–662. Perakakis, P., Joffily, M., Taylor, M., Guerra, P., & Vila, J. (2010). KARDIA: A Matlab software for the analysis of cardiac interbeat intervals. Computer Methods and Programs in Biomedicine, 98, 83–89. https://doi.org/10.1016/j.cmpb.2009.10.002 Pilgrim, J. A. (1994). Psychological aspects of high and low blood pressure. Psychological Medicine, 24, 9–14. https://doi.org/ 10.1017/S0033291700026787 Prospective Studies Collaboration. (2002). Age-specific relevance of usual blood pressure to vascular mortality: A meta-analysis of individual data for one million adults in 61 prospective studies. The Lancet, 360, 1903–1913. https://doi.org/10.1016/ S0140-6736(02)11911-8 Qiu, C., Winblad, B., & Fratiglioni, L. (2005). The age-dependent relation of blood pressure to cognitive function and dementia. Lancet Neurology, 4, 487–499. https://doi.org/10.1016/S1474- 4422(05)70141-1 Qureshi, A. I., Suri, M. F., Kirmani, J. F., & Divani, A. A. (2005). Prevalence and trends of pre-hypertension and hypertension in United States: National Health and Nutrition Examination Surveys 1976 to 2000. Medical Science Monitor, 11 , CR403–CR409. Raaijmakers, E., Faes, T. J., Scholten, R. J., Goovaerts, H. G., & Heethaar, R. M. (1999). A meta-analysis of published studies concerning the validity of thoracic impedance cardiography. Annals of the New York Academy of Sciences, 873, 121 –127. https://doi.org/10.1111/j.1749-6632.1999.tb09458.x Reyes del Paso, G. A. (1994). A program to assess baroreceptor cardiac reflex function. Behaviour Research Methods Instruments & Computers, 26, 62–64. https://doi.org/10.3758/ BF03204567 Reyes del Paso, G. A., González, M. I., & Hernández, J. A. (2010). Comparison of baroreceptor cardiac reflex sensitivity estimates from inter-systolic and ECG R-R intervals. Psychophysiology, 47, 1102–1108. https://doi.org/10.1111/j.1469-8986. 2010.01018.x Reyes del Paso, G. A., González, M. I., Hernández, J. A., Duschek, S., & Gutiérrez, N. (2009). Tonic blood pressure modulated the relationship between baroreceptor cardiac reflex sensitivity and cognitive performance. Psychophysiology, 46, 932–938. https://doi.org/10.1111/j.1469-8986.2009.00832.x Reyes del Paso, G. A., Hernández, J. A., & González, M. I. (2004). Differential analysis in the time domain of the baroreceptor cardiac reflex sensitivity as a function of sequence length. Psychophysiology, 41 , 483–488. https://doi.org/10.1111/ j.1469-8986.2004.00178.x Reyes del Paso, G. A., Langewitz, W., Robles, H., & Perez, N. (1996). A between-subjects comparison of respiratory sinus arrythmia and baroreceptor cardiac reflex sensitivity as noninvasive measures of tonic parasympathetic cardiac control. International Journal of Psychophysiology, 22, 163–171. https:// doi.org/10.1016/0167-8760(96)00020-7 Reyes del Paso, G. A., Langewitz, W., Mulder, L. J. M., van Roon, A., & Duschek, S. (2013). The utility of low frequency heart rate variability as an index of sympathetic cardiac tone: A review with emphasis on a re-analysis of previous studies. Psychophysiology, 50, 477–487. https://doi.org/10.1111/psyp. 12027 Robbe, H., Mulder, L., Ruddel, H., Langewitz, W., Veldman, J., & Mulder, G. (1987). Assessment of BRS by means of spectral analysis. Hypertension, 10, 538–543. Rosengren, A., Tibblin, G., & Wilhelmsen, L. (1993). Low systolic blood pressure and self-perceived wellbeing in middle aged men. British Medical Journal, 306, 243–246. https://doi.org/ 10.1136/bmj.306.6872.243 Sarlo, M., de Zambotti, M., Gallicchio, G., Devigili, A., & Stegagno, L. (2013). Impaired cerebral and systemic hemodynamics under
cognitive load in young hypotensives: A transcranial Doppler study. Journal of Behavioral Medicine, 36, 134–142. https://doi. org/10.1007/s10865-012-9410-8 Schuepbach, D., Boeker, H., Duschek, S., & Hell, D. (2007). Rapid cerebral hemodynamic modulation during mental planning and movement execution: Evidence of time-locked relationship with complex behavior. Clinical Neurophysiology, 118, 2254–2262. https://doi.org/10.1016/j.clinph.2007.07.013 Steptoe, A., & Vögele, C. (1990). Cardiac baroreceptor reflex function during postural change assessed using noninvasive spontaneous sequence analysis in young men. Cardiovascular Research, 24, 627–632. https://doi.org/ 10.1093/cvr/24.8.627 van Roon, A. M., Mulder, L. J. M., Althaus, M., & Mulder, G. (2004). Introducing a baroreflex model for studying cardiovascular effects of mental workload. Psychophysiology, 41 , 961 –981. https://doi.org/10.1111/j.0048-5772.2004.00251.x Vasan, R. S., Larson, M. G., Leip, E. P., Kannel, W. B., & Levy, D. (2001). Assessment of frequency of progression to hypertension in non-hypertensive participants in the Framingham Heart Study: A cohort study. The Lancet, 358, 1682–1686. https://doi. org/10.1016/S0140-6736(01)06710-1 Weisz, N., Schandry, R., Jacobs, A., Mialet, J., & Duschek, S. (2002). Early contingent negative variation of the EEG and attentional flexibility are reduced in hypotension. International Journal of Psychophysiology, 45, 253–260. https://doi.org/ 10.1016/S0167-8760(02)00032-6 Wessely, S., Nickson, J., & Cox, B. (1990). Symptoms of low blood pressure: A population study. British Medical Journal, 301 , 362–365. https://doi.org/10.1136/bmj.301.6748.362 53
Wesseling, K. H., Jansen, J. R., Settels, J. J., & Schreuder, J. J. (1993). Computation of aortic flow from pressure in humans using a nonlinear, three-element model. Journal of Applied Physiology, 74, 2566–2573. Wesseling, K. H., De Wit, B., Van der Hoeven, G. M. A., Van Goudoever, J., & Settels, J. J. (1995). Physiocal, calibrating finger vascular physiology for Finapres. Homeostasis, 36, 67–82. WHO. (1978). Arterial hypertension. In Technical Report Series No. 628. Geneva, Switzerland: World Health Organisation. Yasumasu, T., Reyes del Paso, G. A., Takahara, K., & Nakashima, Y. (2006). Reduced baroreflex cardiac sensitivity predicts increased cognitive performance. Psychophysiology, 43, 41 –45. https://doi.org/10.1111/j.1469-8986.2006.00377.x
Received November 29, 2016 Revision received April 13, 2017 Accepted April 13, 2017 Published online November 21, 2017
Stefan Duschek UMIT –University for Health Sciences, Medical Informatics and Technology Institute of Psychology Eduard-Wallnöfer-Zentrum 1 6060 Hall in Tirol Austria stefan.duschek@umit.at