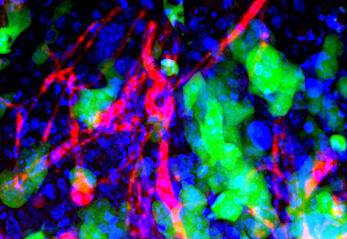
21 minute read
Soyeon (Sophie) Cho '24
Ultrasound Mediated Delivery of erapeutics
BY SOYEON (SOPHIE) CHO '24
Advertisement
Cover Image: A mouse’s brain a er intravenous administration of experimental nanoparticles that can bypass the blood-brain barrier (BBB). Cell nuclei are shown in blue, blood vessels in red, and human cancer cells in green. ese experimental nanoparticles are one of the current therapeutic e orts, such as ultrasound, to administer drugs across the BBB. Image Source: Wikimedia Commons Introduction
Ultrasound is de ned as sound waves with high frequencies that are not audible by humans. Because sound waves are much safer than other methods used for imaging, due to the lack of radiation, ultrasound has been used for diagnostic imaging of fetuses in pregnant women as well as swelling, infection, or other forms of pain in internal organs. Also, unlike x-rays, ultrasound scans clearly indicate so tissues, which explains its widespread use. However, applications of ultrasound outside imaging have been explored in the past several years. Nonimaging uses of ultrasound use the frequencies between the audible region (less than 20 kHz) and the diagnostics ultrasound region (more than 10 MHz) (Mitragotri, 2005). is large range of ultrasound frequencies allows for many di erent uses outside of imaging, including the delivery of therapeutic drugs for patients.
E ects of Ultrasound Cavitation
e direct and indirect e ects of ultrasound waves allow for a wide range of ultrasound applications in therapeutics. e primary e ect is that the ultrasound waves directly interact with mediums like cells and tissues through periodic oscillations of a frequency and amplitude determined by the ultrasound source (Mitragotri, 2005). For the secondary e ects, ultrasound increases temperature as the medium absorbs the oscillating sound waves. is impacts tissues with a higher absorption coe cient for ultrasound waves, such as bones rather than muscle tissue (Suslick, 1988). A more signi cant secondary e ect comes from cavitation, which is the cleavage, growth, and oscillation of microbubbles hit by ultrasound waves.
Microbubbles have a diameter of around 3 µm and are inserted into the site of interest through intravenous injection. In addition to assisting delivery of drugs and gene products, microbubbles can also act as contrast agents for imaging, since they oscillate in response to ultrasound much more than cells (Blomley et al., 2001). Despite initial concerns about having gaseous spaces within the blood vessels, clinical studies have demonstrated no safety hazards from microbubbles, due to their small size (Nanda et al., 1997).
ere are two major types of cavitation, dependent on the stability of the oscillatory motions of the microbubbles. Stable cavitation involves stable,
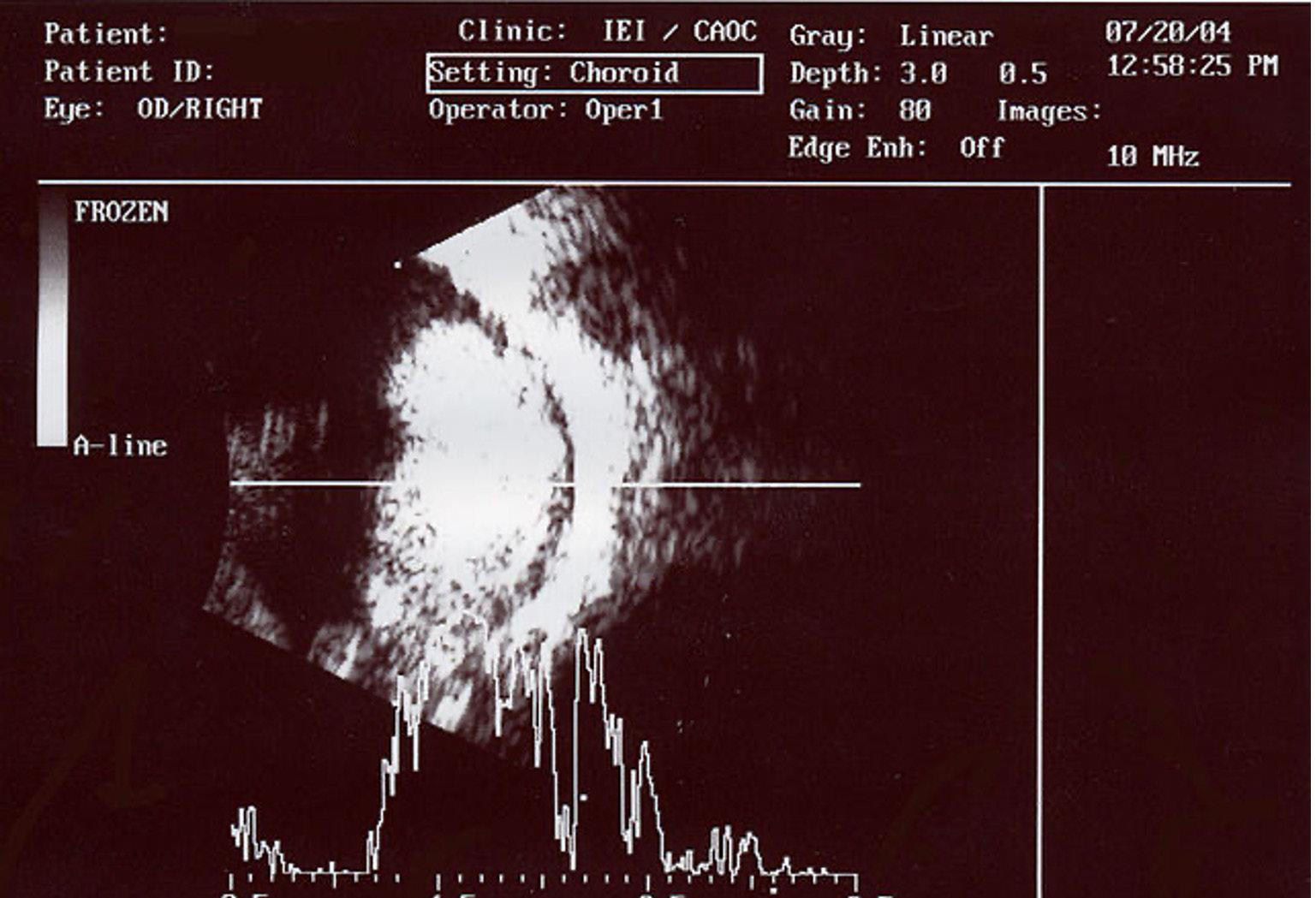
repeating oscillations of the microbubbles, and it is the main mechanism for acoustic streaming. In acoustic streaming, the oscillations produce velocities circling around each microbubble, and for high amplitude oscillations, the velocities induce shear stresses on surrounding tissue (Pitt et al., 2004). ese shear stresses cause the lysis of red blood cells (also called hemolysis) and vesicles like liposomes (Rooney, 1970; Marmottant & Hilgenfeldt, 2003). Acoustic streaming involves microstreaming based on the scale of shear stresses.
In inertial cavitation, microbubbles grow and collapse in irregular patterns, unlike the repeating oscillations in stable cavitation. Because of the collapses, it is also called collapse cavitation. Inertial cavitation is the main mechanism for sonochemistry, shock waves, and liquid microjets. Sonochemistry occurs as the sudden collapse of microbubbles quickly increases temperature in the microbubbles and induces chemical reactions like free radical generation. is step relates sonochemistry to sonodynamic therapy. Shock waves are also caused by the sudden collapse of microbubbles and can induce drug transport, since microbubble collapse disturbs the surrounding tissue and membranes that may regulate the entrance of foreign molecules like drugs. Shock waves both contribute to sonophoresis, also called phonophoresis, and sonoporation, also called transient membrane permeabilization. Sonophoresis is the transdermal delivery of topically applied drugs, and it occurs as shock waves increase the permeability of the skin. Sonoporation is the impermanent damage to the cell membranes and tissues by shock waves, which enhance the delivery of drugs. Liquid microjets are formed by a collapse of a microbubble near the surface, which then cleaves the gas bubble and shoots liquid to the surrounding tissue, also inducing drug transport by causing stress and thus relating to the sonophoresis (Mitragotri, 2005). One consequence is that non-site cells may also undergo lysis due to the microjets hitting them at high velocities (Nyborg, 2001).
Generally, these mechanisms and resulting phenomena, such as sonodynamic therapy, sonophoresis, and sonoporation, disrupt surrounding tissue through oscillations and collapses from the ultrasound-generated microbubbles. ey also contribute to a major type of applications: ultrasound mediated delivery of drugs or gene therapy products. For ultrasound-mediated delivery, microbubbles are injected with the nanoparticles delivered inside of the bubbles, inducing stress to the tissue surrounding the medium and increasing cavitation (Chowdhury et al., 2017). Building on the mechanisms and resulting phenomena for US-mediated delivery of the drugs, this review will discuss the following: di erence between the enhanced uptake of drugs through ultrasound, molecules in US-mediated therapeutics, and other applications including the disruption of the blood-brain barrier (BBB), transdermal drug delivery, and more.
e direct and indirect e ects of ultrasound waves allow for a wide range of ultrasound applications in therapeutics. e primary e ect is
Image 1: An ocular ultrasound of a large retinoblastoma tumor, a malignant tumor in the retina, within the eye of a three-year-old boy. Image Source: Wikimedia Commons
that the ultrasound waves directly interact with mediums like cells and tissues through periodic oscillations of a frequency and amplitude determined by the ultrasound source (Mitragotri, 2005). For the secondary e ects, ultrasound increases temperature as the medium absorbs the oscillating sound waves. is impacts tissues with a higher absorption coe cient for ultrasound waves, such as bones rather than muscle tissue (Suslick, 1988). A more signi cant secondary e ect comes from cavitation, which is the cleavage, growth, and oscillation of microbubbles hit by ultrasound waves.
Microbubbles have a diameter of around 3 µm and are inserted into the site of interest through intravenous injection. In addition to assisting delivery of drugs and gene products, microbubbles can also act as contrast agents for imaging, since they oscillate in response to ultrasound much more than cells (Blomley et al., 2001). Despite initial concerns about having gaseous spaces within the blood vessels, clinical studies have demonstrated no safety hazards from microbubbles, due to their small size (Nanda et al., 1997).
ere are two major types of cavitation, dependent on the stability of the oscillatory motions of the microbubbles. Stable cavitation involves stable, repeating oscillations of the microbubbles, and it is the main mechanism for acoustic streaming. In acoustic streaming, the oscillations produce velocities circling around each microbubble, and for high amplitude oscillations, the velocities induce shear stresses on surrounding tissue (Pitt et al., 2004). ese shear stresses cause the lysis of red blood cells (also called hemolysis) and vesicles like liposomes (Rooney, 1970; Marmottant & Hilgenfeldt, 2003). Acoustic streaming involves microstreaming based on the scale of shear stresses.
In inertial cavitation, microbubbles grow and collapse in irregular patterns, unlike the repeating oscillations in stable cavitation. Because of the collapses, it is also called collapse cavitation. Inertial cavitation is the main mechanism for sonochemistry, shock waves, and liquid microjets. Sonochemistry occurs as the sudden collapse of microbubbles quickly increases temperature in the microbubbles and induces chemical reactions like free radical generation. is step relates sonochemistry to sonodynamic therapy. Shock waves are also caused by the sudden collapse of microbubbles and can induce drug transport, since microbubble collapse disturbs the surrounding tissue and membranes that may regulate the entrance of foreign molecules like drugs. Shock waves both contribute to sonophoresis, also called phonophoresis, and sonoporation, also called transient membrane permeabilization. Sonophoresis is the transdermal delivery of topically applied drugs, and it occurs as shock waves increase the permeability of the skin. Sonoporation is the impermanent damage to the cell membranes and tissues by shock waves, which enhance the delivery of drugs. Liquid microjets are formed by a collapse of a microbubble near the surface, which then cleaves the gas bubble and shoots liquid to the surrounding tissue, also inducing drug transport by causing stress and thus relating to the sonophoresis (Mitragotri, 2005). One consequence is that non-site cells may also undergo lysis due to the microjets hitting them at high velocities (Nyborg, 2001).
Generally, these mechanisms and resulting phenomena, such as sonodynamic therapy, sonophoresis, and sonoporation, disrupt surrounding tissue through oscillations and collapses from the ultrasound-generated microbubbles. ey also contribute to a major type of applications: ultrasound mediated delivery of drugs or gene therapy products. For ultrasound-mediated delivery, microbubbles are injected with the nanoparticles delivered inside of the bubbles, inducing stress to the tissue surrounding the medium and increasing cavitation (Chowdhury et al., 2017). Building on the mechanisms and resulting phenomena for US-mediated delivery of the drugs, this review will discuss the following: di erence between the enhanced uptake of drugs through ultrasound, molecules in US-mediated therapeutics, and other applications including the disruption of the blood-brain barrier (BBB), transdermal drug delivery, and more.
Enhanced Uptake of Drugs rough Ultrasound
Ultrasound enhances the uptake of drugs through two main mechanisms: sonoporation and local release. e rst mechanism, sonoporation, occurs when the shock waves from inertial cavitation temporarily weaken the cell membranes and tissues, which enhances the previously limited delivery of drugs through the membranes (Mitragotri, 2005). Sonoporation is also called transient membrane permeabilization, and its e ects can range from an increase in the permeability of membranes to cell death, microvascular hemorrhage, and rearrangement of tissue structure, depending on intensity (Miller et al., 2002). us, its e ects need to be controlled for when delivering DNA or drugs to a speci c
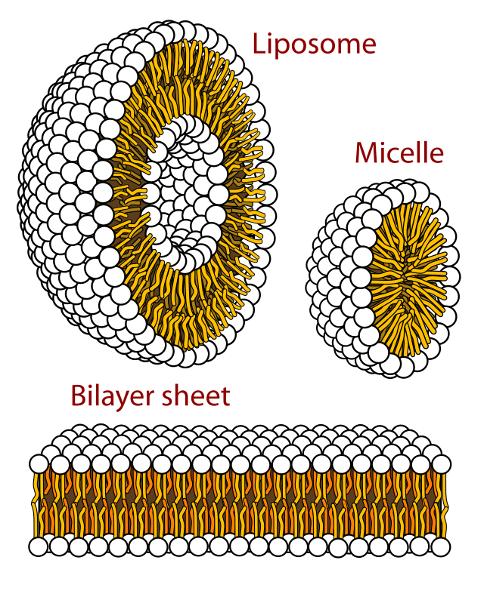
site.
e second mechanism, local release (also known as targeted deliver), delivers drugs inside a carrier vesicle to a site, instead of drugs in their original form (Pitt et al., 2004). Ultrasound is applied to the site of interest, resulting in two major processes of local release of the vesicle. First, the shock waves from inertial cavitation from the microbubble will expand through the uid in the site. If the carrier is close to the source of the shock wave, the shear stress from the shock wave may be enough to rupture the vesicle and release the drug (Sundaram et al., 2003; Marmottant & Hilgenfeldt, 2003). Second, carrier vesicles denser than water, such as liposomes, will be pulled towards the microbubbles through convection; this process is called acoustic pressure. Acoustic pressure will increase the shearing stress applied on the vesicles through shock waves, because the closer the vesicles are to the microbubbles, the higher the shear stress will be (Nyborg, 2001; Guzmán et al., 2003). Because local release can speci cally target the cells (i.e., tumor cells) in a site of interest, it explains the concept of local delivery. Sonoporation and local release are both mechanisms that can jointly increase drug concentrations at the site of interest, while being minimally invasive to the surrounding non-site tissue.
Some other mechanisms have been tested for their e ect on drug uptake. Large scale convection motion from ultrasound beams, rather than repeated oscillations, build convection motion in the vascular system that increases the rate of drug delivery for in vitro systems (Starritt et al., 1989). However, acoustic streaming is less applicable for in vivo systems because the blood vessels already have rapid convection motion, and outside the vascular system, uids are not abundant enough for the convection motion to form (Pitt et al., 2004).
Molecules in Ultrasound Mediated erapeutics
Drugs transported without carriers are called free drugs. Many of the molecules (i.e., drugs, genes) in ultrasound mediated therapeutics are transported in carriers partially because mechanisms like local release work when ultrasound are speci cally applied to carriers. Furthermore, carriers prevent the molecules from interacting with tissue not in the targeted site. One type of carrier is a polymeric carrier, which consists of a polymer with a speci c site that is degraded by extreme pH, aerobic conditions, and enzymes at the site (Howard Jr et al., 1997). Polymeric carriers have been tested in speci c studies, although more work is needed to apply them into wider settings (Pitt et al., 2004).
Another type of carrier includes lipophilic molecules like liposomes or micelles. Liposomes have lipophilic membranes and hydrophilic centers while micelles have lipophilic centers, which allow both to carry lipophilic drugs and only liposomes to encapsulate hydrophilic drugs in the centers (Ning et al., 1994; Husseini et al., 2000; Pitt et al., 2004). As previously mentioned, lipophilic molecules like liposomes and micelles have a higher density than water, meaning they are pulled towards microbubbles through convective motion and more easily disturbed by ultrasound. Out of liposomes, cationic liposomes are more e ective gene carriers because the negatively charged DNA is more rmly bound to the liposomes until the ultrasound-mediated cavitation releases the gene to the speci c site
Image 2: An image demonstrating the structures of a liposome, a micelle, and a bilayer sheet, all of which are phospholipid aqueous solution structures. e white circles represent the hydrophilic heads, and the yellow strands represent the lipophilic tails. Image Source: Wikimedia Commons
Image 3: A schematic image of lipid vesicles, or liposomes, with red hydrophilic heads and black lipophilic tails. e green circles represent the carried particles, which could be neurotransmitters, dye, drugs, or nanoparticles. Image Source: Wikimedia Commons Microbubbles themselves are also e ective carriers for molecules like genes. Gene carrying microbubbles are created by applying ultrasound to a surfactant, or a substance that reduces a liquid’s surface tension, surrounded by gas and the gene to be transferred (Pitt et al., 2004). A er injecting these gene carrying microbubbles into a vessel close to the site, ultrasound is initially applied at low intensity for imaging. Once microbubbles arrive at the site, ultrasound intensity is increased, and inertial cavitation causes microbubbles to collapse and open up pathways through the vessel walls (Price & Kaul, 2002; Miura et al., 2002).
Other Applications
Some other applications of ultrasound in therapeutics include the disruption of the bloodbrain barrier (BBB), which is a semipermeable layer made up of endothelial cells, pericytes, and astrocytes. e BBB prevents the passage of large molecules with a molecular weight larger than 40 kilodaltons between the brain and the surrounding blood vessels; many drugs have a weight larger than 40 kilodaltons, preventing easy movement into the brain (Daneman & Prat, 2015; Mehta et al., 2017). However, following the intravenous injection of microbubbles, focused ultrasound (FUS) at a frequency of around 220,000 times per second can be used to expand and contract the microbubbles in the capillaries, which causes cavitation in the capillaries and disrupts the BBB (Banerjee et al., 2021; Wu et al., 2021). is allows for more routes to delivery drugs, such as modi ed tight junctions through the walls of the capillaries (Burgess et al., 2015). Transdermal drug delivery, or sonophoresis, is another application of ultrasound outside traditional drug delivery. In response to the skin’s layers preventing macromolecules like protein from entering the inner parts of the body, ultrasound is focused on the desired region to permeabilize the skin and allow for drugs to pass the skin into the site. It provides an alternative to drug delivery via needles, especially at frequencies lower than 100 kHz (close to the audible region) (Boucaud et al., 2002). Sonophoresis was rst successfully tested in 1954 for digital polyarthritis (the in ammation and sti ness of ve or more joints) by delivering the hormone hydrocortisone using ultrasound (Fellinger & Schmidt, 1954). e e ects of sonophoresis with higher frequencies (higher than 1 MHz) have been clinically tested and used to deliver drugs like salicylic acid and lidocaine. Other drugs like insulin for diabetes and oligonucleotides have been preclinically tested for skin in ammation (Boucaud et al., 2002). In particular, the delivery of salicylic acid was tested with higher frequencies of larger than 1 MHz, which increased salicylic acid transport when ultrasound was applied for 20 minutes to guinea pigs (Bommannan et al., 1992). Vaccine delivery through sonophoresis has also been explored, given that the skin’s immune cells readily receive the vaccines delivered transdermally (Mitragotri & Kost, 2004).
Conclusion
Di erent types of ultrasound mediated delivery of therapeutics are at varying stages of development. For example, LIPUS treatments for bone fractures and nonunions are in clinical stages, while ultrasound mediated delivery of
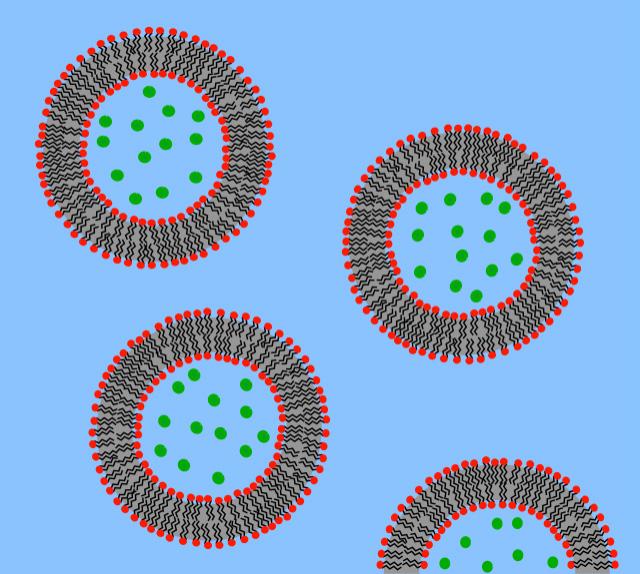
proteins or DNA are still in development stages. For protein delivery, it has mostly focused on the delivery of insulin to address diabetes, as well as some other regulatory hormones (Mitragotri et al., 1995). One major limitation towards transitioning to advanced trials is that it is di cult to determine the appropriate intensity of the ultrasound for therapeutics delivery (Pitt et al., 2004). Ultrasound needs to be strong enough so that protein is transported through the epidermal layer but not too strong so that tissue is not damaged permanently. Furthermore, safety concerns arise from the biological consequences of ultrasound treatments (Nyborg, 2001). Su cient stable or inertial cavitation from the ultrasound may successfully permeabilize the membrane, but also damage the cell functions and going against the purpose of helping cells.
One possible solution is to make the carriers more accepted by the human immune system. Carriers made from native proteins like albumin, a plasma protein made by the liver that transports hormones and enzymes, would appear less foreign and pass the membrane more easily (Shohet et al., 2000; Spada et al., 2021). Also, carriers attached to polymers like poly(ethylene oxide) (PEO) are called polymersomes. ese polymersomes transport macromolecules like liposomes while not stimulating phagocytes in the immune system, and they are more stable than liposomes against thermal transitions (Lee et al., 2001; Li et al., 2006). Similarly, targeting molecules such as antibodies could be bound to microbubbles by the pairing of complementary binders on antibodies and microbubbles (Takalkar et al., 2004). us, the ultrasound intensity needed to transport therapeutics would be lower than carriers without these modi cations. More research on modi cations to carriers or ultrasound intensity would help explore ultrasound mediated delivery of a wider variety of therapeutics. It is anticipated that clinical applications of this technique will greatly a ect how we treat conditions, ranging from diabetes to bone fractures.
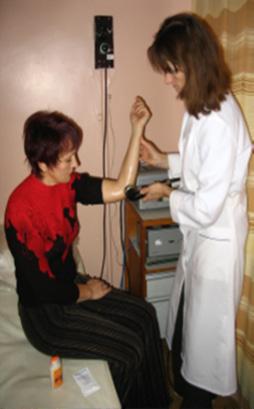
References
Banerjee, K., Núñez, F. J., Haase, S., McClellan, B. L., Faisal, S. M., Carney, S. V., Yu, J., Alghamri, M. S., Asad, A. S., Candia, A. J. N., Varela, M. L., Candol , M., Lowenstein, P. R., & Castro, M. G. (2021). Current Approaches for Glioma Gene erapy and Virotherapy. Frontiers in Molecular Neuroscience, 14, 621831. https://doi. org/10.3389/fnmol.2021.621831
Blomley, M. J. K., Cooke, J. C., & Unger, E. C. (2001). Science, medicine, and the future: Microbubble contrast agents: a new era in ultrasound. BMJ, 322(7296), 1222–1225. https:// doi.org/10.1136/bmj.322.7296.1222
Bommannan, D., Okuyama, H., Stau er, P., & Guy, R. H. (1992). Sonophoresis. I. e use of high-frequency ultrasound to enhance transdermal drug delivery. Pharmaceutical Research, 09(4), 559–564. https://doi. org/10.1023/A:1015808917491
Boucaud, A., Garrigue, M. A., Machet, L., Vaillant, L., & Patat, F. (2002). E ect of sonication parameters on transdermal delivery of insulin to hairless rats. Journal of Controlled Release, 81(1–2), 113–119. https://doi.org/10.1016/S01683659(02)00054-8
Burgess, A., Shah, K., Hough, O., & Hynynen, K. (2015). Focused ultrasound-mediated drug delivery through the blood–brain barrier. Expert Review of Neurotherapeutics, 15(5), 477–491. https://doi.org/10.1586/14737175.2015.1028369
Carvalho, D. C. L., & Cliquet, A. (2004). e Action of Low-intensity Pulsed Ultrasound in Bones of Osteopenic Rats. Arti cial Organs, 28(1), 114–118. https://doi.org/10.1111/j.15251594.2004.07091.x
Chowdhury, S. M., Lee, T., & Willmann, J. K. (2017). Ultrasound-guided drug delivery in
Image 4: An image of a sonophoresis procedure, through which a clinician administers drugs on a patient’s skin and transdermally delivers therapeutics. Image Source: Wikimedia Commons
cancer. Ultrasonography, 36(3), 171–184. https:// doi.org/10.14366/usg.17021
Cook, S. D., Salkeld, S. L., Popich-Patron, L. S., Ryaby, J. P., Jones, D. G., & Barrack, R. L. (2001). Improved Cartilage Repair A er Treatment With Low-Intensity Pulsed Ultrasound: Clinical Orthopaedics and Related Research, 391, S231–S243. https://doi.org/10.1097/00003086200110001-00022
Daneman, R., & Prat, A. (2015). e Blood–Brain Barrier. Cold Spring Harbor Perspectives in Biology, 7(1), a020412. https://doi.org/10.1101/ cshperspect.a020412
Doan, N., Reher, P., Meghji, S., & Harris, M. (1999). In vitro e ects of therapeutic ultrasound on cell proliferation, protein synthesis, and cytokine production by human broblasts, osteoblasts, and monocytes. Journal of Oral and Maxillofacial Surgery, 57(4), 409–419. https:// doi.org/10.1016/S0278-2391(99)90281-1
Fellinger, K. & Schmidt, J. Klinik and therapies des chromischen gelenkreumatismus. Maudrich Vienna, Austria 549–552 (1954).
Guzmán, H. R., McNamara, A. J., Nguyen, D. X., & Prausnitz, M. R. (2003). Bioe ects caused by changes in acoustic cavitation bubble density and cell concentration: A uni ed explanation based on cell-to-bubble ratio and blast radius. Ultrasound in Medicine & Biology, 29(8), 1211–1222. https:// doi.org/10.1016/S0301-5629(03)00899-8
Howard, W. A., Bayomi, A., Natarajan, E., Aziza, M. A., El-Ahmady, O., Grissom, C. B., & West, F. G. (1997). Sonolysis Promotes Indirect Co−C Bond Cleavage of Alkylcob(III)alamin Bioconjugates. Bioconjugate Chemistry, 8(4), 498–502. https://doi.org/10.1021/bc970077l
Husseini, G. A., El-Fayoumi, R. I., O’Neill, K. L., Rapoport, N. Y., & Pitt, W. G. (2000). DNA damage induced by micellar-delivered doxorubicin and ultrasound: Comet assay study. Cancer Letters, 154(2), 211–216. https://doi.org/10.1016/S03043835(00)00399-2
Ilyashenko, I. (2009). Phonophoresis procedure. Wikimedia Commons. Wikimedia Commons. https://upload.wikimedia.org/wikipedia/commo ns/9/93/%D0%A4%D0%BE%D0%BD%D0%BE %D1%84%D0%BE%D1%80%D0%B5%D0%B7. png.
Koch, S., Pohl, P., Cobet, U., & Rainov, N. G. (2000). Ultrasound enhancement of liposomemediated cell transfection is caused by cavitation e ects. Ultrasound in Medicine & Biology, 26(5), 897–903. https://doi.org/10.1016/S03015629(00)00200-3
Lee, J. C.-M., Bermudez, H., Discher, B. M., Sheehan, M. A., Won, Y.-Y., Bates, F. S., & Discher, D. E. (2001). Preparation, stability, and in vitro performance of vesicles made with diblock copolymers. Biotechnology and Bioengineering, 73(2), 135–145. https://doi.org/10.1002/bit.1045
Li, L., AbuBaker, O., & Shao, Z. J. (2006). Characterization of Poly(Ethylene Oxide) as a Drug Carrier in Hot-Melt Extrusion. Drug Development and Industrial Pharmacy, 32(8), 991–1002. https://doi. org/10.1080/03639040600559057
Marmottant, P., & Hilgenfeldt, S. (2003). Controlled vesicle deformation and lysis by single oscillating bubbles. Nature, 423(6936), 153–156. https://doi.org/10.1038/nature01613
MDougM. (2007). Lipid vesicles. Wikimedia Commons. Wikimedia Commons. https:// commons.wikimedia.org/wiki/File:Lipid_ vesicles.svg.
Mehta, A. M., Sonabend, A. M., & Bruce, J. N. (2017). Convection-Enhanced Delivery. Neurotherapeutics, 14(2), 358–371. https://doi. org/10.1007/s13311-017-0520-4
Miller, D. L., Pislaru, S. V., & Greenleaf, J. F. (2002). [No title found]. Somatic Cell and Molecular Genetics, 27(1/6), 115–134. https:// doi.org/10.1023/A:1022983907223
Mitragotri, S. (2005). Healing sound: e use of ultrasound in drug delivery and other therapeutic applications. Nature Reviews Drug Discovery, 4(3), 255–260. https://doi.org/10.1038/nrd1662
Mitragotri, S., Blankschtein, D., & Langer, R. (1995). Ultrasound-Mediated Transdermal Protein Delivery. Science, 269(5225), 850–853. https://doi.org/10.1126/science.7638603
Mitragotri, S., & Kost, J. (2004). Low-frequency sonophoresis. Advanced Drug Delivery Reviews, 56(5), 589–601. https://doi.org/10.1016/j. addr.2003.10.024
Miura, S., Tachibana, K., Okamoto, T., & Saku, K. (2002). In vitro transfer of antisense oligodeoxynucleotides into coronary endothelial
cells by ultrasound. Biochemical and Biophysical Research Communications, 298(4), 587–590. https://doi.org/10.1016/S0006-291X(02)02467-1
Mourad, P. D., Lazar, D. A., Curra, F. P., Mohr, B. C., Andrus, K. C., Avellino, A. M., McNutt, L. D., Crum, L. A., & Kliot, M. (2001). Ultrasound Accelerates Functional Recovery a er Peripheral Nerve Damage. Neurosurgery, 48(5), 1136–1141. https://doi.org/10.1097/00006123-20010500000035
Nanda, N. C., & Carstensen, E. L. (1997). Echoenhancing agents: Safety. In N. C. Nanda, R. Schlief, & B. B. Goldberg (Eds.), Advances in Echo Imaging Using Contrast Enhancement (pp. 115–131). Springer Netherlands. https://doi. org/10.1007/978-94-011-5704-9_6
Natebw. (2006). Retinoblastoma ultrasound. Wikimedia Commons. Wikimedia Commons. https://commons.wikimedia.org/wiki/ File:Retinoblastoma_ultrasound.jpg.
Ning, S., Macleod, K., Abra, R. M., Huang, A. H., & Hahn, G. M. (1994). Hyperthermia induces doxorubicin release from long-circulating liposomes and enhances their anti-tumor e cacy. International Journal of Radiation Oncology*Biology*Physics, 29(4), 827–834. https://doi.org/10.1016/0360-3016(94)90572-X
Nyborg, W. L. (2001). Biological e ects of ultrasound: Development of safety guidelines. Part II: General review. Ultrasound in Medicine & Biology, 27(3), 301–333. https://doi.org/10.1016/ S0301-5629(00)00333-1
Pitt, W. G., Husseini, G. A., & Staples, B. J. (2004). Ultrasonic drug delivery – a general review. Expert Opinion on Drug Delivery, 1(1), 37–56. https://doi.org/10.1517/17425247.1.1.37
Price, R. J., & Kaul, S. (2002). Contrast Ultrasound Targeted Drug and Gene Delivery: An Update on a New erapeutic Modality. Journal of Cardiovascular Pharmacology and erapeutics, 7(3), 171–180. https://doi. org/10.1177/107424840200700307
Rooney, J. A. (1970). Hemolysis Near an Ultrasonically Pulsating Gas Bubble. Science, 169(3948), 869–871. https://doi.org/10.1126/ science.169.3948.869
Shohet, R. V., Chen, S., Zhou, Y.-T., Wang, Z., Meidell, R. S., Unger, R. H., & Grayburn, P. A. (2000). Echocardiographic Destruction of Albumin Microbubbles Directs Gene Delivery to the Myocardium. Circulation, 101(22), 2554–2556. https://doi.org/10.1161/01. CIR.101.22.2554
Spada, A., Emami, J., Tuszynski, J. A., & Lavasanifar, A. (2021). e Uniqueness of Albumin as a Carrier in Nanodrug Delivery. Molecular Pharmaceutics, 18(5), 1862–1894. https://doi. org/10.1021/acs.molpharmaceut.1c00046
Starritt, H. C., Duck, F. A., & Humphrey, V. F. (1989). An experimental investigation of streaming in pulsed diagnostic ultrasound beams. Ultrasound in Medicine & Biology, 15(4), 363–373. https://doi.org/10.1016/03015629(89)90048-3
Suslick, K. S. (Ed.). (1988). Ultrasound: Its chemical, physical, and biological e ects. VCH.
Takalkar, A. M., Klibanov, A. L., Rychak, J. J., Lindner, J. R., & Ley, K. (2004). Binding and detachment dynamics of microbubbles targeted to P-selectin under controlled shear ow. Journal of Controlled Release, 96(3), 473–482. https:// doi.org/10.1016/j.jconrel.2004.03.002
Villarreal, M. R. (2007). Phospholipids aqueous solution structures. Wikimedia Commons. Wikimedia Commons. https://commons. wikimedia.org/wiki/File:Phospholipids_ aqueous_solution_structures.svg.
Watanabe, Y., Matsushita, T., Bhandari, M., Zdero, R., & Schemitsch, E. H. (2010). Ultrasound for Fracture Healing: Current Evidence. Journal of Orthopaedic Trauma, 24(Supplement 1), S56–S61. https://doi.org/10.1097/BOT.0b013e3181d2efaf
Wu, S.-K., Tsai, C.-L., Huang, Y., & Hynynen, K. (2020). Focused Ultrasound and MicrobubblesMediated Drug Delivery to Brain Tumor. Pharmaceutics, 13(1), 15. https://doi. org/10.3390/pharmaceutics13010015
Wyatt, E., & Davis, M. (2017). Nanoparticles in Brain Metastases. Wikimedia Commons. Wikimedia Commons. https://commons. wikimedia.org/wiki/File:Nanoparticles_in_ Brain_Metastases_(26155288848).jpg.