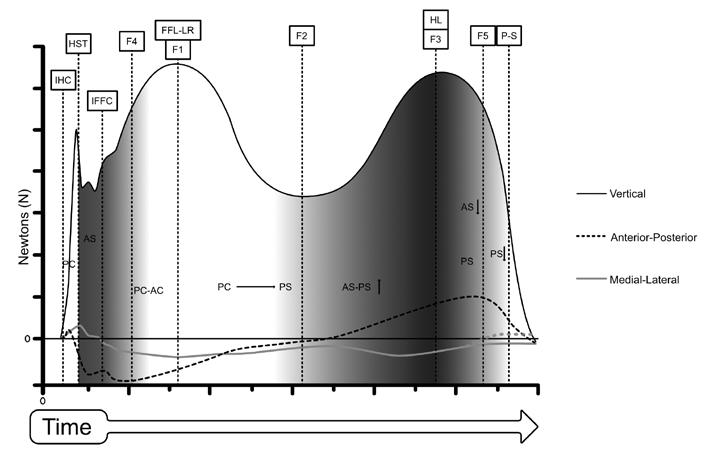
12 minute read
4 page CPD article
Essentials of Biomechanics
Principles of gait: Understand the Principles of the Acceleration Lever System
Advertisement
By Andy Horwood
Visiting Lecturer & Fellow Staffordshire University
INTRODUCTION
The foot must create cycles of compliance and stiffening throughout the stance phase of gait to safely manage its exposure to collision-induced energies. These forces derive from initial contact, full body weight loading, body weight transfer over the foot, and the application of acceleration power. Foot compliance allows energy dissipation (shock absorption), while stiffness permits energy storage followed by its release and application for locomotive power against the ground. Both properties require adaptable levels of elasticity to set differing foot flexibility during gait (see figure 1). Adaptability in the mechanical properties of a foot at any moment derives primarily from muscular activity and the gait speed, actions that both alter connective tissue tension and structural shape changes across the pedal anatomy. Together, these factors should set the foot up for terminal stance ankle plantarflexion power application to provide appropriate acceleration onto the next step
Figure 1: The cycles of foot compliance and stiffening occurring during stance phase are applied to impulses generated over a force-time curve. Paler areas indicating periods of compliance and darkening areas indicating increasing or decreasing stiffness across the foot vault. IHC = initial heel contact, HST = heel strike transient, IFFC = initial forefoot contact, FFL-LR = forefoot loading completed at loading response, HL = heel lift, and P-S = pre-swing. Vertical impulse peaks and troughs identified as F1, F2, and F3. Horizontal antero-posterior impulse peaks demonstrated by F4 and F5 peaks.
Image from the upcoming text ‘Origins and Principles of Clinical Biomechanics in Human Locomotion’, with permission of www.healthystep.co.uk.
The heel fat pad provides compliance at initial heel contact (IHC) during the heel strike transient (HST). Heel contact is followed by active foot stiffening across the vault (arch), which continues until initial forefoot contact (IFFC) when the foot starts to increase its compliance to dissipate the forefoot loading collision forces to the end of loading response (FFL-LR). The end of loading response creates the F1 vertical peak in vertical force as the foot reaches its foot-flat plantigrade posture. Throughout early midstance the foot remains compliant as muscle activity within the foot reduces to allow energy dissipation and increasing ground contact until the trough in vertical forces at F2. This compliance permits the foot to lengthen and widen by expressing linear-elastically during a period of lowering forces. However, towards the end of its stretching range, connective tissue becomes exponentially stiffened, thus demonstrating classic viscoelastic properties (Stolwijk et al, 2014; Bjelopetrovich and Barrios, 2016; Takabayashi et al, 2020). As late midstance progresses, the foot’s connective tissues become increasingly stiffened across the vault to a point of peak normal pronation as defined by Horwood and Chockalingam (2017), prior to heel lift (HL). The ability to create a large F3 peak of vertical force and the F5 peak of posterior force for acceleration, relies on the foot becoming a stiffened acceleration platform, thus reducing is ability to dissipate energy at the heel lift boundary. Pre-swing (P-S) is a period of increasing compliance readying the foot for swing phase. Note the F4 and F5 peaks of horizontal anteroposterior forces at the start and end of stance as the lower limb drives forces forwards into the ground, and then posteriorly into the ground behind the body.
Lever systems are very simple machines that biology crudely uses to create motion at joints. They consist of point of motion (a fulcrum usually provide by a joint), a site of power application (an effort point provided by muscles), resistance (a load being moved, consisting of a certain region of body mass), and a beam (usually provided by a single long bone within land vertebrates) that is used to provide interaction between all these features (see figure 2). You will probably remember from your school days, that there are three lever systems based on where the fulcrum (pivot point), resistance (load), and effort are positioned in relation to each other. A class one system has the fulcrum in the middle, a class two has the resistance in the middle, and the class three has the effort in the middle (see figures 2, 3, and 4). The mechanical efficiency of any lever system is dictated by the distance the resistance is positioned from the fulcrum compared to the effort’s distance from the fulcrum
Figure 2: Components of a lever arm consist of a beam, a point of rotation known as the fulcrum (F), a resistance (R) being moved or resisted, and the effort (E) that apply the forces against the resistance. Each component is demonstrated here within a class two lever, where the effort is applied further away from the fulcrum than the resistance. Such an arrangement requires less effort force to move or resist the resistance force.
Image from the upcoming text ‘Origins and Principles of Clinical Biomechanics in Human Locomotion’, with permission of www.healthystep.co.uk.
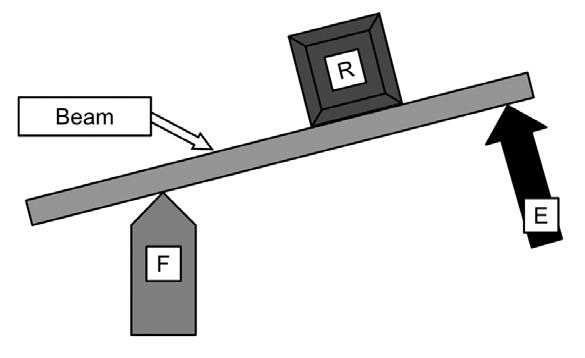
A class one lever has the fulcrum in the middle, like a seesaw. This means either the effort and resistance can be the same distance from the fulcrum on opposing sides, or one can be nearer to the fulcrum than the other across the fulcrum. Whichever is further from the fulcrum has the mechanical advantage (see figure 3). Humans use crude class one lever systems around the hip concurrently with class three levers, but more for stabilisation of hip joints in the frontal plane rather than for significant sagittal plane joint motion. For large ranges of joint motion in the sagittal plane (flexions and extensions), class three levers are preferred. These place the resistance further away from the joint than the effort, so they are mechanically inefficient. The reason they are used in biology ties into concepts of muscle physiology costs. Changing muscle fibre length is metabolically expensive, especially when concentrically shortening them. Thus, reducing the amount of change necessary in muscle fibre length during locomotion overrides the mechanically efficiency of the lever system when it comes to gait energetics. Thus, applying the effort point of the quadriceps close to the knee via the patella and its tendon (ligament) means that the quadriceps only need to contract a little to create a large extension rotation of the knee that moves the foot and ankle a long way during late swing phase (see figure 4). This arrangement dramatically decreases the metabolic costs of power generation and motion, although it requires greater effort.
Figure 3: The distance that the effort (E - arrows) and resistance (R) are positioned away from the fulcrum (F) affects the efficiency of a lever system. Here, within a class one lever system such as a seesaw [A], E and R can be equal distance from the fulcrum. If the effort force matches the resistance forces, the system is balance [B], but if E lies further from F than does the R [C], E has the advantage. The reverse is true in [D], where R is given the advantage by being further from F.
Image from the upcoming text ‘Origins and Principles of Clinical Biomechanics in Human Locomotion’, with permission of www.healthystep.co.uk.
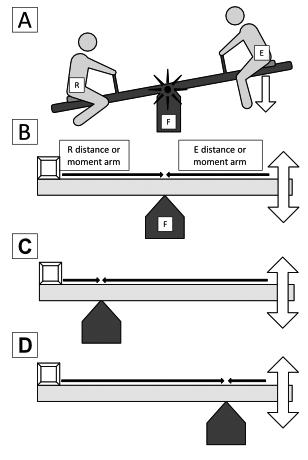
The Type Two Lever Arm of Acceleration
There is only really one class two lever system used within the foot during gait (and it is arguable one). It is utilised during the action of initial heel lift. There is also another arguably class two lever system used at the hip via gluteus maximus resisting initial contact hip flexion that controls the drop of the upper body’s centre of mass (CoM) during loading response. This system also raises the CoM forwards by initiating hip extension in the sagittal plane at the start of midstance. However, it’s the one at heel lift that has the greatest influence on the foot ability to influence gait and its own healthy function. At heel lift, effort is applied to the posterior surface of the calcaneus via the Achilles tendon enthesis. The resistance being moved is the mass of the lower limb needing to be lifted from the ground and tipped forward to follow the CoM of the, head, arms, and trunk.
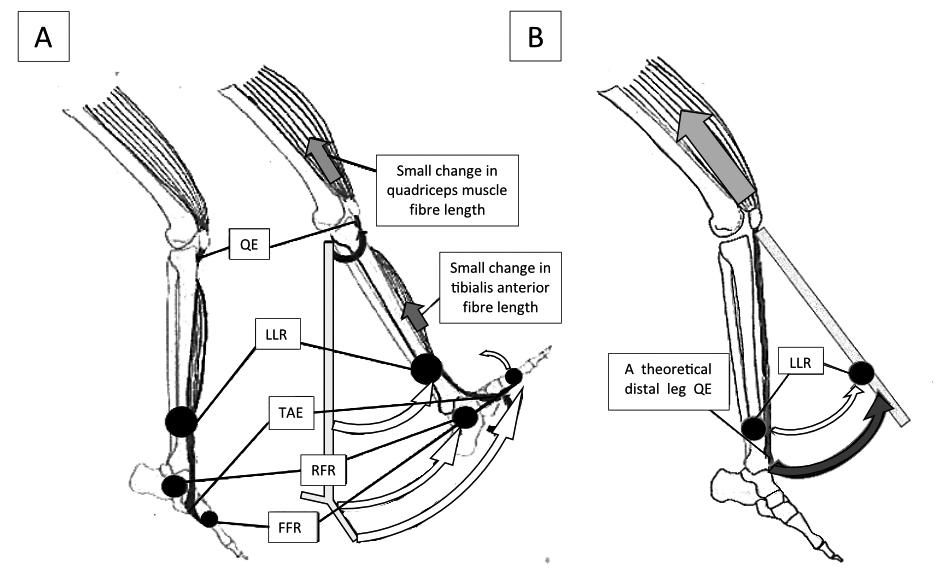
Figure 4: Muscle fibre length changes are metabolically expensive activities. During swing phase, tibialis anteriorinduced ankle dorsiflexion motion and large ranges of quadriceps-induced knee extension motion are achieved with only small muscle fibre length changes. This is because tendinous attachment points are located very close to the joint axis that they cross (A). If the quadriceps attached to the distal tibia (B), then extensive muscle fibre shortening would be required to bring about knee extension and such motion would be applied at a considerably slower rate.
Image from the upcoming text ‘Origins and Principles of Clinical Biomechanics in Human Locomotion’, with permission of www.healthystep.co.uk.
The trunk should already be positioned anterior to the foot before heel lift, being dragged forward behind the swing limb and falling forward under centrifugal and gravitational forces. Thus, the body’s CoM should not require heel lift power to continue to progress forwards and downwards. However, a powerful heel lift improves gait energetics. The fulcrum at heel lift is not the ankle, for although the ankle undergoes plantarflexion motion at this time, the fulcrum point lies within the forefoot where the plantarflexion power is being applied to the ground. Ideally the fulcrum point should lie within the metatarsophalangeal (MTP) joints. Medial MTP joints make better fulcrums that lateral ones for reasons that will be discussed in later articles. If the foot functions efficiently, the resistance of the lower limb mass should lie between the effort (Achilles attachment) and the fulcrum (MTP joints), lying over the midfoot. This is much like the situation that occurs in emptying a wheelbarrow. Like a wheelbarrow (see figure 5), where the load lies within the barrow (or over the midfoot) dictates how easy it will be to tip the contents (resistance of body mass) forward over the fulcrum (MTP joins) and onto the next footstep during terminal stance. Obviously, the beam-like barrow must be ‘solid’ for the effort on the handles to move the load.
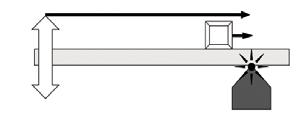
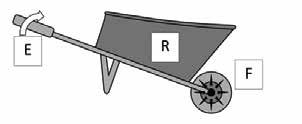
Figure 5: The classic example of a class two lever is the emptying a wheelbarrow, acting as a beam. Effort (E) is applied at the handle, the resistance (R) is within and above the barrow, and the fulcrum (F) is the wheel that is allowing the barrow to rotate. This arrangement can be used to represent the Achilles attachment as E, body mass being moved above the foot as R, with the length of the foot to the metatarsophalangeal joints providing the beam. The metatarsophalangeal joints form the fulcrum of rotation. The closer R (represented as the box in the lower image) is to the fulcrum (black star), and the further E is from the fulcrum the easier it is to tip the beam forward. The foot, if turned into a semistiffened beam can act as a type two lever for acceleration during heel lift, but muscular and connective tissue stiffening of the foot must occur for the foot to behave in a beam-like manner.
Image from the upcoming text ‘Origins and Principles of Clinical Biomechanics in Human Locomotion’, with permission of www.healthystep.co.uk.
Summary
Although class three levers are preferred in biology, as they use minimal muscle fibre length changes to produce faster and greater ranges of motion for low metabolic costs, there are exceptions. These are when mechanical advantage potentially improves energetics over muscular metabolic costs because stabilisation is more important than motion, or power derives from other sources than active muscle fibre length changes. Class one levers within the lower limb are used for stabilising the hip within the frontal plane, particularly during single limb support. A biomechanically unusual class two lever system is used for heel lift at the starts of the acceleration phase of gait. This is probably possible because plantarflexion power is derived from elastic recoil from the energy stored within the Achilles tendon during late midstance, rather than via active muscular contraction within triceps surae. The lever system is debatably because it requires the foot to be semi-stiffened across the vault, which in turn necessitates activity of muscles such as tibialis posterior, peroneus longus, and other plantar extrinsic muscles and intrinsic muscles that are working on other lever systems at the same time (Ferris et al, 1995; Kokubo et al, 2012; Farris et al, 2019; 2020). Thus, the effort required for stable, efficient heel lift involves more than the Achilles power alone.
REFERENCES:
Bjelopetrovich A, Barrios JA. (2016). Effects of incremental ambulatory-range loading on arch height index parameters. Journal of Biomechanics. 49(14): 3555-3558.
Farris DJ, Kelly LA, Cresswell AG, Lichtwark GA. (2019). The functional importance of human foot muscles for bipedal locomotion. Proceedings of the National Academy of Sciences of the United States of America. 116(5): 1645-1650.
Farris DJ, Birch J, Kelly L. (2020). Foot stiffening during the push-off phase of human walking is linked to active muscle contraction, and not the windlass mechanism. Journal of the Royal Society: Interface. 17(168): 20200208.
Ferris L, Sharkey NA, Smith TS, Matthews DK. (1995). Influence of extrinsic plantar flexors on forefoot loading during heel rise. Foot & Ankle International. 16(8): 464-473.
Horwood AM, Chockalingam N. (2017). Defining excessive, over, or hyper-pronation: A quandary. The Foot. 31: 49-55.
Kokubo T, Hashimoto T, Nagura T, Nakamura T, Suda Y, Matsumoto H, et al. (2012). Effect of the posterior tibial and peroneus longus on the mechanical properties of the foot arch. Foot & Ankle International. 33(4): 320-325.
Stolwijk NM, Koenraadt KLM, Louwerens JWK, Grim D, Duysens J, Keijsers NLW. (2014). Foot lengthening and shortening during gait: A parameter to investigate foot function? Gait & Posture. 39(2): 773-777.
Takabayashi T, Edama M, Inai T, Nakamura E, Kubo M. (2020). Effect of gender and load conditions on foot arch height index and flexibility in Japanese youths. Journal of Foot & Ankle Surgery. 59(6): 1144-1147.
In the next issue: Essentials of Biomechanics, the complications of using the foot as a beam for a class two lever system are discussed.
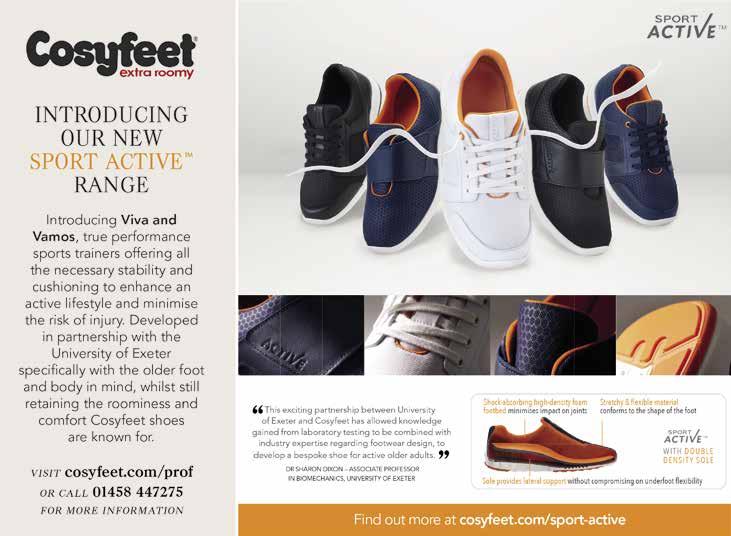