35 minute read
Review of Nitrification and Distribution System Water Quality—Frederick Bloetscher and Daniel E
FWRJ Review of Nitrification and Distribution System Water Quality
Frederick Bloetscher and Daniel E. Meeroff
The goal of water utilities is to provide highquality drinking water that does not pose a public health concern, and to provide sufficient quantities of that water when needed to their customers (Bloetscher, 2011). An adequate and safe potable water supply is a key requirement for a stable society; however, the provision of safe drinking water is a public trust issue—the public trusts the utility system’s ability to deliver adequate supplies of safe drinking water. The expectation of water systems in the United States and Canada is that they will operate 24 hours a day, uninterrupted, and provide safe, clean drinking water for all who need it (Bloetscher, 2008).
When these expectations are not met, problems with public trust will likely occur; Flint, Mich., is a prime example. Rarely does the public understand what is required to meet these expectations, which is a credit to the effectiveness to which these systems have been designed and operated for well over 100 years. The proof is that, in the U.S., for the most part, publicly owned water and sewer systems comply with all regulations on a consistent basis, but the backlog of infrastructure needs means that longer-term compliance may become an issue. Biofilms are one of the issues that can complicate regulatory compliance.
Biofilms Defined
Biofilms result from microorganisms, which are the most widely distributed life forms on the planet (Chapelle, 1993). They are known to inhabit and thrive in the presence of moisture and nutrients, both of which exist in plentiful supplies in water distribution networks, where microorganisms can grow in the form of biofilms (Videla, 1996).
Biofilms are complex aggregates of microorganisms embedded in a highly hydrated extracellular matrix that show structural heterogeneity resulting from a diverse and complex microcosm. Biofilms are often observed as an unwanted accumulation attached to a surface, such as the inner wall of a water distribution pipeline. During biofilm growth, microorganisms excrete extracellular polymeric substances, which lead to the formation of a slime layer that connects cells and anchors them to the surface and to each other. From the microbial perspective, biofilms provide an ideal habitat as a source of nutrients, oxygen stratification, resistance to velocity currents, and protection from grazers and biocides (Videla, 1996).
From the utility perspective, the undesirable accumulation of biofilms with actively growing slime layers can lead to biofouling. If left uncontrolled, biofilms can catalyze the formation of calcium carbonate deposits that restrict the effective pipe diameter and become a considerable issue for water distribution systems, particularly with regard to hygienic, operational, and economic consequences.
Pathogenic microorganisms have been isolated from biofilms, including viruses, fungi, yeast, protozoa (such as amoebae and ciliates), invertebrates, and microbial toxins (Eboigbodin et al., 2008; Bachmann and Edyvean, 2006; Meeroff et al., 2019; and references therein). The presence of these microorganisms in potable water distribution systems represents a potential public health threat. Specifically, release of pathogens harbored in biofilms can lead to an increase in the incidence of gastrointestinal symptoms from waterborne infections caused by bacterial, viral, and parasitic microorganisms. The Centers for Disease Control and Prevention identified biofilms as the source for 65 percent of human bacterial infections from community water supply-associated outbreaks (EPA, 2008c). Gofti et al. (1999) reported epidemiological evidence that children showed close to four digestive problems per person per year and one episode of diarrhea per person per year, attributable to pathogens that developed in the water transmission network after centralized disinfection.
According to the World Health Organization, diseases associated with unsafe water distribution, sanitation, and hygiene cause approximately 1.7 million deaths per year (Prentice, 2002). Mature biofilms in drinking water distribution systems are a highly diverse potential source of human pathogens. A wide range of primary pathogens (i.e., that cause disease in healthy individuals) and opportunistic pathogens (i.e., that cause disease in individuals with underlying conditions that may facilitate infection) have demonstrated the ability to survive and thrive in biofilms.
Primary pathogens, opportunistic pathogens and indicator organisms, including Clostridium (Emde et al., 1992), E. coli (Emde et al., 1992; Geldreich, 1996; Sartory and Holmes, 1997), Enterobacter (LeChevallier et al., 1987; Emde et al., 1992; Geldreich, 1996; Sartory and Holmes, 1997; Lee and Kim, 2003), Legionella (Murga et al., 2001), Pseudomonas (LeChevallier et al., 1987; Emde et al., 1992; Geldreich, 1996; Norton and LeChevallier, 2000; Lee and Kim, 2003), and Staphylococcus (Gelreich, 1996; Lee and Kim, 2003), among others (see Bloetscher et al., 2010; Meeroff et al., 2019), have been reported in biofilms collected from water distribution networks.
An important point to consider is that only coliforms are routinely analyzed for in drinking water, as mandated under the Total Coliform Rule (TCR) and the Groundwater Rule of the Safe Drinking Water Act (SDWA); however, the pathogens and opportunistic pathogens are not.
Table 1 summarizes some of the bacteria typically found in a biofilm.
In addition to health effects resulting from pathogens, biofilms can also contribute to taste, odor, and color issues, which may lead to operational changes at the treatment plant or for the transmission network. Biofilms may also compromise the proper enumeration of indicator organisms and weaken pipe integrity by microbially influenced corrosion (MIC), which is defined as a microbially mediated electrochemical process that permits the onset and acceleration of corrosion (Videla, 1996). Once mature colonies are established, the effects of MIC are often seen.
Microbes cause corrosion directly through metabolic processes that form corrosive chemical species, such as ammonia, hydrogen sulfide, sulfate, ferric, or manganic chlorides (Dillon, 1995). Within the community structure of the biofilm, sulfur can be reduced by anaerobic bacteria to release hydrogen sulfide, which can significantly increase the susceptibility of the pipe to pitting. At the same time, any aerobic bacteria present in the biofilm can corrode metals directly via oxidation. The heterotrophic biomass typically found in a biofilm is supported by the
Frederick Bloetscher, Ph.D., P.E., and Daniel E. Meeroff, Ph.D., E.I., are professors at Florida Atlantic University in Boca Raton.
synergistic effects of mixed growth rates, mixed metabolisms, and high surface-area-to-volume ratios, which allow the biofilm to remain active within the relatively hostile conditions of a pipe environment.
For water distribution systems constructed with metallic materials, corrosion and corrosion control are ongoing issues that, if not addressed, can result in pipe damage/failure, premature aging/replacement, clogging, and increased maintenance requirements. Proper corrosion control has also been shown to increase disinfection effectiveness on biofilms in iron pipes (Le Chevallier et al., 1990).
This all raises the question: Can the existence of biofilms be verified in the water distribution system, and if so, where does it come from and what can be done about it?
Ideal Conditions for Biofilm Growth
Biofilm growth in water distribution systems occurs when microorganisms attach themselves to the pipe walls. The steps in the life cycle of a biofilm include attachment, slime formation, growth, and detachment, or sloughing (Videla, 1996). Key requirements for biofilm development include an active microbial community and interaction with pipe materials (Videla, 1996). The first microorganisms to attach are called “pioneers,” which are most commonly facultative anaerobes that excrete a mass of extracellular polysaccharides, forming the foundation for colonization and further growth.
Fleming and Wingender (2001) estimated that 50 to 90 percent of the biofilm matrix was extracellular material, while only 25 percent was cell matter. Through microbially mediated redox mechanisms, micronutrients are released within this growing layer, which attracts other species. As the biofilm continues to coat the pipe surface, acid formers can reduce the pH near the pipe wall and accelerate corrosion (Videla, 1996). This phenomenon can create localized anodes and, in conjunction with abiotic reactions caused by dissimilar metals or pipe defects, can lead to a steady cathodic deterioration over time.
As the biofilm matures, it grows thicker from the diverse community of microorganisms attracted to the biofilm and from the accumulation of particles that stick to the extracellular polymer matrix. Once a critical thickness is achieved, a depleted oxygen layer forms near the wall and an anaerobic environment, in which sulfatereducing bacteria (SRB) proliferate because the transport of oxygen into the anaerobic layer of the biofilm is limited by the biological activity in the upper layers, is created. The appearance of SRB is indicative of mature biofilm growth, but the biofilm cannot continue to increase in thickness indefinitely. The bulk fluid velocity will act to reduce the thickness of the biofilm on the pipe wall as a result of friction and shear. Periodically, portions of the growing mass become detached (sloughing), releasing bacteria into the bulk fluid. Sloughing can also occur if the system is disturbed by changes in the water velocity, water quality, or water hammer. Sloughing spreads the biofilm.
A variety of factors are known to affect biofilm development in distribution networks, including environmental parameters (pH, temperature, dissolved oxygen, etc.), water quality (nutrients, inorganics, dissolved organic carbon, etc.), pipe materials, system hydraulic regime (stagnant conditions), corrosion control measures, presence of a disinfectant residual, and sediment accumulation (Meeroff et al., 2019; and references therein). Most of the data on the factors that influence biofilm development are based on changes in total viable counts (e.g., heterotrophic plate count) or on changes in the growth of specific microorganisms (e.g., total coliforms). Although a number of comprehensive review articles have been published (Geldreich, 1996; Geldreich and LeChevallier, 1999; Batté et al., 2003; Bachmann and Edyvean, 2006), the interaction among these factors is complex and variable, making predictions difficult.
Currently, researchers lack techniques for effective detection, diagnosis, and control of biofilms. Low pH, low hardness, high chlorides, high sulfates, and a ratio of chlorides to bicarbonate above 0.3 all indicate a greater potential for corrosion and biofouling (Geldreich, 1996). One effective diagnostic tool involves the heterotrophic plate count (HPC) test. Samples that yield HPC counts of more than 500 colonyforming units (CFU)/100 mL and have chlorine residuals less than 0.2 mg/L typically indicate stagnant water or conditions that promote biofilm growth (Geldreich, 1996). Since HPC is nonselective, biofilm material can be collected from a pipe and cultured to isolate specific organisms or their deoxyribonucleic acid (DNA) signatures (Meeroff et al., 2019). For instance, persistent coliform levels may indicate extensive biofilm shedding (Crozes and Cushing, 2000). Biofilms can become a point source of coliforms, leading to TCR violations; therefore, mechanisms for controlling biofilms may be of benefit to reducing coliform levels, as well as other opportunistic pathogens.
Nitrification in the Water Distribution System
Another result of biofilms is nitrification. Nitrite and nitrate are produced during nitrification through ammonia utilization by nitrifying bacteria found in the biofilms. According to the U.S. Environmental Protection Agency (EPA, 2002), the microbial process for nitrification involves two steps.
The first step is the reduction of nitrogen compounds (primarily ammonia), which are sequentially oxidized to nitrite and then nitrate. The nitrification process is primarily accomplished by two groups of autotrophic nitrifying bacteria that can build organic molecules using energy obtained from inorganic sources; in this case, ammonia or nitrite. In the first step of nitrification, ammonia-oxidizing bacteria convert ammonia to nitrite, according to equation 1.
NH3 + O2 → NO2 - + 3H+ + 2e- (1)
Nitrosomonas is the most frequently identified genus associated with this step, although other genera, including Nitrosococcus, and Nitrosospira, can perform this biologically mediated step. Some subgenera, Nitrosolobus and Nitrosovibrio, can also autotrophically oxidize ammonia (Watson et al., 1981).
In the second step of the process, nitriteoxidizing bacteria convert nitrite to nitrate, according to equation 2 (EPA, 2002).
NO2- + H2O → NO3 - + 2H+ +2e- (2)
Nitrobacter is the most frequently identified genus associated with this second step, although other genera, including Nitrospina, Nitrococcus, and Nitrospira, can also autotrophically oxidize nitrite (Watson et al., 1981). Various groups of heterotrophic bacteria and fungi can also carry out nitrification, although at a slower rate than autotrophic organisms (Verstraete and Alexander, 1973; Watson et al., 1981). Speciation of nitrifying bacteria in drinking water systems (Wolfe, 1990 and 2001) suggests that the number of heterotrophic nitrifiers in those systems may be negligible compared to autotrophic nitrifiers.
According to equations 1 and 2, for every mole of ammonia-N produced, a 1-mole equivalent of nitrite-N is produced. Subsequently, for every mole of nitrite-N produced, a 1-mole equivalent of nitrate-N is produced (EPA, 2002). Ammonia can also be released from chloramines through a series of complex reactions. Reactions 2 through 6 in Table 2 describe five mechanisms of ammonia release presented by Woolschlager et al. (2001). According to Valentine et al. (1998), the overall net stoichiometries can be used to examine the relationship between chloramine decay and ammonia production.
It should be noted that nitrifying bacteria are obligate aerobic organisms commonly found in terrestrial and aquatic environments (Holt et al., 1995; Watson et al., 1981). Their growth rates are controlled by: substrate (ammonia-N) concentration, temperature, pH, light, oxygen concentration, and microbial community composition (EPA, 2002). These issues are discussed further.
Under the SDWA, primary maximum contaminant levels (MCLs) have been established for nitrite-N, nitrate-N, and the sum of nitrite-N plus nitrate-N. The MCLs are 1 mg/L for nitrite-N,
Table 2. Overview of Nitrification and Chloramine Reactions (from Woolschlager et al., 2001)
Reaction Description Reaction Eq.
Ammonium and nitrate utilization NH3 + O2 → NO2 - + 3H+ + 2eNO2 - + H2O → NO3 - + 2H+ +2e(1) (2) Release of ammonia through chloramine decay 3NH2Cl → N2 + NH3 + 3H+ +3Cl- (3) Release of ammonium through oxidation of organic matter by chloramine 10NH2Cl + 9H2O + 10C3H7O2N → HCO3 - + 4CO2 +Cl-+ 11NH4 (4) Release of ammonium through oxidation of organic matter by chloramine NH2Cl + 2H+ + 2Fe+3 → 2Fe+3 + NH4 + +Cl- (5) Release of ammonia through catalysis reactions of chloramine at pipe surfaces 3NH2Cl → N2 + NH3 + 3H+ +3Cl- (6) Release of ammonia through oxidation of nitrate by chloramine NH2Cl + H2O + NO2 - → NH3 +HCl + NO3 - (7) 10 mg/L for nitrate-N, and 10 mg/L for nitrite + nitrate (as N). The current nitrite and nitrate standards are measured at the point of entry to the distribution system, so any subsequent elevated nitrite/nitrate levels resulting from nitrification within the distribution system are not identified by compliance monitoring.
Sources of Ammonia, Nitrate, and Nitrite
Excess nitrogen in the form of ammonia in finished water can be the principal cause of nitrification, since ammonia serves as the primary substrate in the nitrification process. Natural sources of nitrogen generally have minimal impacts on water supply distribution systems because the concentration of nitrite-nitrogen in surface water and groundwater is normally far below 0.1 mg/L (Sawyer and McCarty, 1978).
Ammonia occurs naturally in some groundwater supplies, and groundwater can become contaminated with nitrogen as agriculture runoff or fertilizer percolates into aquifers. In south Florida, ammonia is common and can be well above 1 mg/L in some groundwaters. Furthermore, ammonia is deliberately added to a chloraminated water supply. Chloramination is commonly used for secondary disinfection purposes to control microbial growth in finished water disinfection byproducts during chlorination. This is particularly an issue for water systems that have warm water (>60°F) and high background levels of organics. Chloramines include monochloramine, dichloramine, trichloramine, and organochloramines. For disinfection purposes, monochloramine and dichloramine are the preferential forms. In systems utilizing chloramination, the concentration of free ammonia present in the distributed water will be a function of the chlorine-to-ammonia-N (Cl2:NH3-N) ratio. Free ammonia is almost completely eliminated when a 4.67:1 weight ratio of Cl2:NH3-N is used (Kirmeyer et al., 1993, 1995, 2000). Chloramination is typical in south Florida.
Of interest is that the Groundwater Rule applies when water sources have no ammonia in the raw water (quenching is not permitted by the federal rules). This rule permits reduced monitoring if 4-logs of virus removal can be demonstrated. Regulatory agencies encourage this compliance; however, where utilities have ammonia, the 4-log guidelines do not apply. As a result, the argument to chlorinate the ammonia “out of the water,” free chlorinate the water, and then add ammonia back in to create chloramines (monochloramine) does not comply with federal drinking water standards. The nitrogen does not disappear—it’s just combined with chlorine and adds “food” to the nitrogenconsuming bacteria. As a nutrient, the nitrogen is still present and would potentially be available to be used as a nutrient by bacteria. As a result,
6.5 7.0 7.5 8.0 8.5 pH mg N Oxidized/mg TKN/hr
2.0 1.8 1.6 1.4 1.2 1.0 0.8 0.6 0.4 0.2 0.0
6.0 6.5 7.0 7.5 8.0 8.5 pH
Figure 1. pH and oxidation rate versus pH for Nitrosomonas and Nitrobacter (from Grady and Lim, 1980)
the 4-log Groundwater Rule has the unintended consequence of acting as a source of ammonia to the water distribution network.
Natural Organic Material
Song (1999) documented the impact of natural organic material (NOM) on chloramine decay by altering NOM concentrations through granular activated carbon (GAC) adsorption of the test water. Figure 1 shows the impact of NOM on chloramine decay rates. Bone et al. (1999) hypothesized that the NOM oxidation mechanism is the dominant pathway for chloramine decay early in the decay process (i.e., within 24 hours), and that autodecomposition is the dominant cause of chloramine decay later. It should be noted that GAC removal of organics is compromised when the organic content has a relatively high contribution of humic material, which describes the situation in south Florida (Ødegaard et al., 2010).
pH and Alkalinity
Water pH is an important factor in nitrification activity for two reasons. First, a reduction of total alkalinity may accompany nitrification because a significant amount of bicarbonate is consumed in the conversion of ammonia to nitrite. A model developed by Gujer and Jenkins (1974) indicates that 8.64 mg/L of bicarbonate (HCO3 -) will be utilized for each mg/L of ammonia-nitrogen oxidized. While reduction in alkalinity does not impose a direct public health impact, reductions in alkalinity can cause reductions in buffering capacity, which can impact pH stability and corrosivity of the water toward lead and copper, as well as biogeochemically induced deposition of calcium carbonate that might clog the pipeline.
Secondly, nitrifying bacteria are sensitive to pH. Nitrosomonas has an optimal pH between approximately 7 and 8, and the optimum pH range for Nitrobacter is approximately 7.5 to 8. Some utilities have reported that an increase in pH (to greater than 9) can be used to reduce the occurrence of nitrification (Skadsen et al., 1996). According to Wilczak (2001), pH appears to be the most important factor controlling the rate of chloramine autodecomposition. Thomas (1987) stated that the rate of chloramine decay approximately doubles for a drop in pH of 0.7 units. In Florida, Cates and Lavinder (1999) noted that raising pH reduced nitrification. Figure 1 shows that a pH above 8.7 limits nitrification activity.
Monitoring
According to Wilczak et al. (1996), nitrification is often indirectly identified by detection via monitoring the following (EPA, 2002): S Residual disinfectant levels. Low levels of residual disinfectant can allow bacteria in the biofilm to multiply. When residual disinfectant drops below the baseline, nitrification may soon follow; therefore, monitoring and mapping levels of residual disinfectant prove to be a quick and inexpensive tool to pinpoint affected areas and focus mitigation measures. S Nitrite and nitrate levels. Monitoring nitrite and nitrate levels can signal when preventative action is needed. Systems are required to sample for nitrate and nitrite quarterly in the distribution system. More-frequent monitoring may be beneficial in identifying trends and action trigger levels. S Ammonia levels. Ammonia is food for nitrifying bacteria; if ammonia levels are decreasing in at least part of the distribution system, nitrification could be the cause. If the ammonia levels in the system are less than the ammonia levels of the water leaving the treatment plant as finished water, or less than the system’s baseline ammonia levels, then nitrification may be occurring. Monitoring will determine baseline ammonia levels for the system, and then a closer review of data from any system location for decreasing ammonia levels might trigger mitigation action. S Dissolved oxygen levels. A noticeable decrease in dissolved oxygen concentration may indicate bacteria are consuming oxygen
via the nitrification process during the biologically mediated conversion from ammonia to nitrate. S pH and alkalinity. (as noted previously) S Temperature change. An increase in water temperature is an indirect indicator of biological activity; higher temperatures facilitate higher bacterial growth rates. S HPC counts. Frequently, but not always, systems that have nitrification occurring may also have increases in HPCs, coliform-positive test results, or both, since the conditions that are favorable to indicator microorganisms are the same as those favorable to the nitrogenoxidizing microorganisms.
If these issues occur, nitrification is suggested, and a more-active control strategy must be implemented.
Example of Results From Distribution System Samples
Tables 3 and 4 outline the results from a series of samples for the water system studied. These results indicate that the conditions are such as to favor nitrifying bacteria, although none were specifically identified. Of greater concern is the presence of Pseudomonas species.
Nitrification Control Strategies
Nitrification can degrade water quality, and the formation of nitrite/nitrate and disinfection byproduct (DBP) formation during nitrification mitigation are the only water quality issues identified in the literature with the potential to impact public health directly. The problem is greatest when temperatures are warm and water demand is low; however, there are multiple effective means to control nitrification issues.
Nutrient Control
Biofilms are more difficult to remove from pipelines once established, and therefore, they must be monitored and controlled. Many Continued on page 14 Florida Water Resources Journal • August 2022 13
Continued from page 13 different methods have been used to control biofilms; however, in most circumstances, biofilm control requires the use of a variety of tools, and the relative effectiveness is typically sitespecific. In general, biofilms can be managed by removing organic matter and nutrients during water treatment, inactivation of microorganisms via sensible use of disinfectants and residuals, pH control and proper distribution system maintenance practices (i.e., flushing, avoiding stagnant conditions, minimizing corrosion of iron pipe surfaces, and managing contamination from external sources).
Nutrient suppression involves controlling the source of assimilable organic carbon (AOC), or phosphorus and nitrogen. This can be accomplished by GAC, enhanced coagulation, preozonation, or membrane processes; unfortunately, several months are required before impacts of nutrient control can actually be observed in the system. Rather than reduce the phosphorus levels, which are rarely limiting in water distribution networks, phosphate can be added (less than 1.1 mg/L P) to sequester iron to inhibit corrosion (Batté et al., 2003). In practice, however, this method leads to either a limited change in microbial distributions or an increase in biomass.
pH Control
A major factor that is often overlooked is that the pH of the water going into the water distribution system may encourage nitrification bacterial growth. Nitrosomonas has an optimal pH between approximately 7 and 8, and the optimum pH range for Nitrobacter is approximately 7.5 to 8. To reduce the potential for growth in water distribution systems with lime softening plants, the appropriate lime dose should be used and adjusted only to the extent that the finished water enters the distribution system at a pH above 8.7, and preferably around 9. This is relatively straightforward to accomplish for groundwater treated by lime softening, but for nanofiltration plants, the pH is usually under 7, so preventing aggressive water and keeping the pH below 8 would be an appropriate goal.
Disinfection
A common strategy for disinfection is chemical control or treatment of nitrifying bacteria. Such treatment typically involves either the maintenance of high distribution system disinfectant residuals (greater than 2 mg/L) or periodic breakpoint chlorination. Analytical survey results of ten U.S. utilities showed that greater than 90 percent of distribution system samples with increased nitrite and nitrate levels, indicative of nitrification, occurred in water with disinfectant residuals less than 2 mg/L (Wilczak et al., 1996). Many utilities have found that increasing disinfectant residuals by increasing chemical doses or managing water age has helped to control nitrification.
Utilities can use booster chlorination in the distribution system to increase disinfectant residuals. This practice is generally not employed in chloraminated distribution systems because chloramines are normally more stable than free chlorine (Woolschlager et al., 2001; Valentine et al., 1998). In addition, uncontrolled blending of chlorinated and chloraminated water could occur near a chlorine booster station; in some cases, uncontrolled blending has been shown to cause unintended breakpoint chlorination, increases in DBP levels, or decreases in disinfectant residuals (Mahmood et al., 1999; Muylwyk et al., 1999).
The key to stopping nitrification is to starve the nitrifying bacteria of nitrogen. The most effective way to do this is to temporarily convert disinfectant from chloramine to free chlorine. Free chlorine is more effective at inactivating ammonia-oxidizing bacteria colonies than chloramines (Wolfe et al., 1990). As a result, breakpoint chlorination is also used by utilities to treat nitrifying bacteria. Nitrification mitigation techniques, such as breakpoint chlorination or temporarily switching from chloramines to free chlorine, can result in increased levels of DBPs regulated under the Stage 1 Disinfectants and Disinfection Byproduct Rule. The EPA suggests that DBP samples collected during a nitrification mitigation episode not be included in MCL compliance calculations, and it specifically allows short-term exceedance of maximum residual disinfectant levels (MRDLs) to control microbiological contamination problems.
Because biofilms provide a barrier to mass transfer, there are diffusion-limited zones within the thickness of the film in which microorganisms are exposed to sublethal doses, leading to increased survivability and resistance. Once a biofilm is established, it may take a high level of chlorine residual (>0.2-1.0 mg/L) to reduce microbial levels appreciably (Le Chevallier et al., 1990). It may take one to two months of continuous chlorination to eradicate all biofilmassociated biomass and desorbable organics at a dose of 3.5 mg/L Cl2, with a residual of 0.1-0.2 mg/L Cl2 (Fass et al., 2003).
Superchlorination in problematic areas isolated from the rest of the network can be an effective approach, but high chlorine doses may not be recommended in situations that require precise control of DBPs and pipe corrosion. Chloramines can be an effective alternative because they are capable of deeper penetration into the biofilm, but they are less reactive (LeChevallier et al., 1990; Bachmann and Edyvean, 2006). Other options include alternative disinfectants (e.g., hydrogen peroxide or ultraviolet [UV]), pH adjustment, membrane processes, or Cu or Ag ionization units).
Distribution System Maintenance
Distribution system maintenance is vitally important in controlling biofilms. From a hydrodynamic perspective, transmission networks should be designed and operated to minimize sediment accumulation, microbial adhesion, and regrowth. During stagnant flow conditions or during periods of low demand, conditions will be favorable for biofilm establishment. It’s precisely in these areas of the system, where nutrients can accumulate, that ferrous materials provide fresh surfaces for colonization and disinfectant residuals can become rapidly depleted.
Routine systematic flushing (annually or semi-annually) is a primary component of proper
Table 3. Bacteria Isolated in Water Samples
Bacteria Isolated Site 1 Site 2 Site 3 Site 4 Site 5 Site 6
Bacilllus sp. x x x x Pseudomonoa fluorescens x x x x x Pseudomonas aeruginosa x Enterobacter cloacae x x x Atypicla mycobacterium x x x x x Candida Yeast x x x x Micrococcus sp. x x Staphylococcus sp. (not S. aures) x x x Enterobacter cloacae x x Steno. maltophilia x x Agrobacteriaum radiobacter x 14 August 2022 • Florida Water Resources Journal
distribution system maintenance, although it does not prevent recolonization (Walker and Morales, 1997). Flushing and/or pigging (use of a water-propelled device), along with valve turning at regular intervals, are typically used in practice. Specific subsections of a distribution system can be isolated and flushed with high velocities to discourage biofilm growth. Shock chlorination may also be part of a targeted flushing process. Dead zones (resulting in excessive hydraulic residence times) can be eliminated by valve exercising and eliminating excess storage, while low-flow areas can be eliminated by line resizing (Crozes and Cushing, 2000) or routing flow to fire hydrants. Eboigbodin et al. (2008) noted that temperature and velocity also play a major role in biofilm formation. Continual positive pressure is also recommended (Kirmeyer et al., 2000) and is a best available technology (BAT) in the TCR.
Niquette et al. (2000) demonstrated that proper pipe material selection is important in biofilm control. They noted that polyvinyl chloride (PVC) and polyethylene had the least potential to form biofilms, although polyethylene may promote the growth of Legionella more than PVC (van der Kooij and Veenendaal, 2001). They also noted that ferrous materials were more likely to form biofilms than any other material. Cloete et al. (2003) reported similar findings, demonstrating that galvanized iron had the least resistance to biofilm formation among the materials they tested. Asbestos concrete and cast iron showed similar biofilm formation potential.
They found PVC to have more resistance than the other materials; thus, changing pipe materials is another control option, and nonferrous materials should be used whenever possible. If ferrous materials must be used, coatings (e.g., cement lining) can be used to discourage fouling and biofilm corrosion. Unfortunately, existing pipelines cannot easily be replaced or lined. In these cases, treatment of the problem in situ may be the next best option.
According to Schrempp et al. (1994), mechanically cleaning pipelines; draining and cleaning reservoirs; and dead-end, unidirectional, and continuous flushing were not sufficient to control nitrification at one Midwestern utility. When these strategies were replaced with breakpoint chlorination, nitrification was controlled, and target residuals could be maintained. Some systems using breakpoint chlorination have reported an initial increase in HPC bacteria and total coliform levels immediately following treatment, which is probably attributable to biofilm sloughing (Odell et al., 1996; Wilczak et al., 1996).
Replace Aging Infrastructure
Corroding pipes and equipment provide plenty of crevices for nitrifying bacteria to
Bacteria Isolated Swab 1 Swab 2 Swab 3 Swab 4 Swab 5
Swab 6t
Bacilllus x x x x x x Pseudomonoa fluorescens x x x x x x Atypical mycobacterium x Candida Yeast x x x Steno. maltophilia x
escape exposure to residual disinfectant. If excessive maintenance is required to keep the infrastructure clean, then replacement of the problematic components with newer, less corrodible equipment should be considered.
Reduce Water Age
Nitrification will usually show up first in areas where residence time (or “water age”) is highest; for example, dead-end mains, storage tanks, and areas where pressure planes overlap. These areas should be monitored carefully for biofilm development. Disinfectant levels drop when water stands still in the system. If water usage drops, a temporary solution is to flush mains to keep new water moving (TCEQ, 2017). Pipe looping also helps to alleviate water age. Water conservation strategies can exacerbate water age issues, since, in most cases, the pipes are oversized for potable water service.
Preventive Maintenance
Utilities should include measures to reduce biofilms in their regularly scheduled maintenance: S Some systems have found that a hard flush once a year helps to keep nitrification in check. S Especially with cast iron pipes, mechanical pigging can remove deposits, where nitrifying bacteria can establish colonies. S Some systems find it necessary to temporarily convert to free chlorine disinfection as part of a periodic preventive maintenance routine.
Conclusions
To determine the potential for biofilms to be present, even before they create water distribution impacts, an analysis of the water system should be undertaken. In the sample case presented here, the results suggested that nitrification was present, but no specific species were found in the samples. A major factor was that the pH of the water going into the water distribution system favored nitrification bacterial growth.
To reduce the potential for growth in water distribution systems for lime softening plants, the appropriate lime dose should be used and adjusted only to the extent that the finished water enters the distribution system at a pH above 8.7, and preferably around 9. The lack of flow (indicative of low water use and closed valves) encourages nitrification. The use of rechlorination stations should be reviewed (Barrett et al., 1985; Potts, 2001). The use of chloramines, while needed to maintain a residual, likewise adds ammonia. The ammonia provides a substrate for these bacteria, especially if the chlorination dose has combined with the raw ammonia to achieve 4-log removal under the Groundwater Rule.
Finally, if ammonia is present in the raw water, the 4-log virus rule does not apply. Trying to extinguish the ammonia is not permitted under the federal rules, and only adds nitrogen to the water, which provides food for nitrification bacteria. Pipe materials, maintenance, flushing programs, and monitoring are ongoing processes that can help minimize the potential for nitrification in the water distribution system.
References
• Bachmann, R. T. and Edyvean, R. G. J. (2006). Biofouling: an historic and contemporary review of its causes, consequences and control in drinking water distribution systems. Biofilms, 2(3): 197-227. • Barrett, S.E., M.K. Davis, and M.J. McGuire. 1985. Blending Chloraminated and Chlorinated Waters. Journal AWWA (1): 50-61. • Batte, M., Mathieu, L., Laurent, P., and Prevost, M. (2003). Influence of phosphate and disinfection on the composition of biofilms produced from drinking water, as measured by fluorescence in situ hybridization. Can. J. Microbiol. 49, 741. • Bloetscher, F. 2011. Utility Management for Water and Wastewater Operators. America Water Works Association, Denver, Colo. • Bloetscher, F., Daniel E. Meeroff, Ph.D., Sharon Long, Ph.D., P.E., ND. Ongoing Issues in Water Distribution Systems and Solutions to Address Them. Science Letters, SL/2021/0294. • Bloetscher, F., Meeroff, D. E., Pisani, J. V., & Long, S. C. (2010). Resolving problematic biofilms Continued on page 16 Florida Water Resources Journal • August 2022 15
Continued from page 15 in buildings and compounds. Environmental
Engineering Science, 27(9), 767-776. • Bloetscher, F. 2008. Water Basics for Decision
Makers: What Local Officials Need to Know about Water and Wastewater Systems. America
Water Works Association, Denver, Colo. • Bone C.C., Harringtom G.W., Oldenburg
P.S., Noguera D.R. Ammonia Release from Chloramine Decay: Implications for the Prevention of Nitrification Episodes.
Proceedings of AWWA Annual Conference,
Chicago, Ill., 1999. Grady, C.P.L, Jr., and H.C.
Lim, 1980. Biological Wastewater Treatment.
Marcel Dekker Inc., N.Y. • Cates, J. and Lavinder, S. 1999. Improving
Chloramine Residuals and Minimizing
Nitrification. Florida Water Resources Journal, 1999, v:2. 26-28. • Chapelle, Francis H. (1993), Groundwater
Microbiology and Geochemistry. John Wiley and
Sons, New York, N.Y. • Cloete, T.E.; Westaard, D.; and Van Vuuren,
S.J. (2002), Biofilm Formation in Hot Water
Systems. Water Science and Technology, 46:9:95. • Crozes, G.F., and Cushing, R.S. (2000).
Evaluating Biological Regrowth in Distribution
Systems. AwwaRF, Denver, Colo. • Dillon, C.P. (1995), Corrosion Resistance of
Stainless Steels. Marcel Dekker Inc., New York,
N.Y. • Eboigbodin, Kevin E.; Seth, Allyson; and Biggs,
Catherine A., A Review of Biofilms in Domestic
Plumbing. Journal AWWA, 100:10, pp 131-138. • Emde, K.M.E., Smith, D.W., and Facey, R. (1992). Initial investigation of microbially influenced corrosion (MIC) in low temperature water distribution systems. Water Research, 26(2): 169-175. • Fass, S., Block, J.-C., Boualam, M., Gauthier,
V., Gatel, D., Cavard, J., Benabdallah, S. and
Lahoussine, V. (2003). Release of organic matter in a discontinuously chlorinated drinking waternetwork. Water Research, 37(3): 493–500. • Flemming, H.C. and Wingender, J. (2001)
Relevance of Microbial Extracellular Polymeric
Substances (EPSs) – Part 1: Structural and Ecological Aspects. Water Science and
Technology, 43:6:1. • Galli, E.; Silver, S.; and Withot, B. (1992).
Pseudomonas: Molecular Biology and
Biotechnology. American Society of
Microbiology, Washington, D.C. • Geldreich, E.E. (1996). Microbial Quality of
Water Supply in Distribution Systems. CRC
Press, Boca Raton, Fla. • Geldreich, E.E., and LeChevallier, M. (1999).
Microbiological quality control in distribution systems. Chapter 18. pp. 18.1-18.49. In: Water
Quality and Treatment (5th ed.). Letterman, RD (ed.). McGraw-Hill Inc. New York, N.Y. • Gofti, L., Balducci, F., Gratacap-Cavailler, B.,
Joret, J.C., Ferley, J.P., and Zmirou, D. (1999).
Waterborne microbiological risk assessment, epidemiological validation of dose–response functions for viruses and protozoans.
Epidemiology, 4: S56 (abstract). • Gujer W., and D. Jenkins, 1974. A Nitrification
Model for Contact Stabilization Activated
Sludge Process. Water Res., 9(5):5. • Holt, David, Rory D. Todd, Anaick Delanoue, and Jennifer S. Colbourne. 1995. A Study of
Nitrite Formation and Control in Chloraminated
Distribution Systems. In Proc. 1995 AWWA
Water Quality Technology Conference; Part II.
AWWA, Denver, Colo. • Kirmeyer, G.J., M. Friedman, J. Clement, A.
Sandvig, P.F. Noran, K.D. Martel, D. Smith, M.
LeChevallier, C. Volk, E. Antoun, D. Hiltebrand,
J. Dyksen, and R. Cushing. 2000. Guidance
Manual for Maintaining Distribution System
Water Quality. AwwaRF and AWWA, Denver,
Colo. • Kirmeyer, G. J., Odell, L. H.; Jacangelo, J.; Wilczak,
A. and Wolfe. R. 1995. Nitrification Occurrence and Control in Chloraminated Water Systems.
AwwaRF and AWWA, Denver, Colo. • Kirmeyer, G., W. Richards, and C.D. Smith. 1994.
An Assessment of Water Distribution Systems and Associated Research Needs. AwwaRF,
Denver, Colo. • Kirmeyer, G.J., G.W. Foust, G. L. Pierson, J.J.
Simmler, M.W. LeChevallier. 1993. Optimizing
Chloramine Treatment. AwwaRF and AWWA,
Denver Colo. • LeChevallier, M.W., Lowry, C.D., and Lee, R.G. (1990). Disinfection of biofilms in a model distribution system. Journal AWWA, 82(7):8799. • Lee, D.-G. and Kim, S.J. (2003). Bacterial species in biofilm cultivated from the end of the Seoul water distribution system. Journal of Applied
Microbiology, 95(2): 317-324. • Mahmood, F., J. Pimblett, N. Grace and B.
Utne. 1999. Combining Multiple Water Sources and Disinfectants: Options for Water Quality
Compatibility in Distribution Systems. In
Proc. 1999 AWWA Water Quality Technology
Conference, Tampa, Fla.: AWWA. Prepared by
AWWA with assistance from Economic and
Engineering Services Inc. 15. • Meeroff, D.E., Shaha, B., Bloetscher, F., Esiobu,
N., Mercer, B., McCorquordale, D., Kari, R. and
Bennett, M., 2019. Characterization of biofilms and mineralogical scale in underground injection well disposal of landfill leachate and industrial wastewater streams. Journal of
Geoscience and Environment Protection, 7(11), p.69. • Murga R., Forster, T.S., Brown, E., Pruckler,
J.M., Fields, B.S., and Donlan, R.M. (2001).
Role of biofilms in the survival of Legionella pneumophila in a model potable water system.
Microbiology, 147(11): 3121-3126. • Muylwyk, Q., A.L. Smith and J.A. MacDonald. 1999. Implications on Disinfection Regime
When Joining Water Systems: A Case Study of Blending Chlorinated and Chloraminated
Water. In Proc. 1999 AWWA Water Quality
Technology Conference, Tampa, Fla.: AWWA. • Norton, C.D. and Le Chevallier, M.W. (2000).
A pilot study of bacteriological population changes through potable water treatment and distribution. Applied and Environmental
Microbiology, 66(1): 268-276. • Niquitte, P.: Servais, P. and Savoir, R. (2000),
Impacts of Pipe Materials on Densities of
Fixed Bacterial Biomass in Drinking Water
Distribution System, Water Research, 34:6:1952. • Odell, Lee H., Gregory J. Kirmeyer, Andrzej
Wilczak, Joseph G. Jacangelo, Joseph P.
Marcinko, and Roy L. Wolfe. 1996. Controlling
Nitrification in Chloraminated Systems. Journal
AWWA, 88(7):86-98. • Ødegaard, H. Østerhus, S.; Melin, E., and B.
Eikebrokk, 2010 NOM removal technologies –
Norwegian experiences, Drink. Water Eng. Sci., 3, 1–9, 2010. • Potts, D.E., W.G. Williams, C.G. Hitz. 2001. A
Satellite Chloramine Booster Station: Design and Water Chemistry. In Proc. 2001 AWWA.
Distribution System Symposium, San Diego,
Calif.: AWWA. • Prentice, T. (2002). Overview. In: Murray C,
Lopez A, editors. The World Health Report 2002: Reducing risks, promoting healthy life.
Geneva: World Health Organization. pp 7 – 14. • Sartory, D.P. and Holmes, P. (1997). Chlorine sensitivity of environmental, distribution system, and biofilm coliforms. Water Science and Technology, 35(11-12): 289-292. • Sawyer, C.N., and P.L. McCarty. 1978. Chemistry for Environmental Engineering, 3rd edition,
McGraw-Hill, NY. *Stage I DBP Rule FACA
Meeting, 2000. • Schrempp, Tom, Ron Goold, Bennet Kwan and Darshan Sarai. 1994. Effect of Mechanical
Cleaning and Free Residual Chlorine on the
Chlorine Demand and Nitrification Process in a Chloraminated System. In Proc. 1994 AWWA
Water Quality Technology Conference; Part II.
San Francisco, Calif.: AWWA. • Skadsen, J. and Larry Sanford. 1996. The
Effectiveness of High pH for Control of
Nitrification and the Impact of Ozone on
Nitrification Control. In Proc. 1996 AWWA
Water Quality Technology Conference. Boston,
Mass.: AWWA. • Song, Daniel J., Ali Sheikholeslami, Linnea
L. Hoover, Kathrin A. Turner, H. Hubert Lai, and Andrzej Wilczak. 1999. Improvement of
Chloramine Stability Through pH Control,
TOC Reduction and Blending at EBMUD,
California. In 1999 AWWA Annual Conference
Proceedings. Chicago, Ill.: AWWA. • Thomas P.M. “Formation and Decay of
Monochloramine in South Australian Water
Supply Systems,” 12th Federal Convention,
Australian Water and Wastewater Association,
Adelaide, Australia, p.268-276, 1987. • TCEQ, 2017 Controlling Nitrification in Public
Water Systems with Chloramines, TCEQ,
Austin, Texas, https://www.tceq.texas.gov/ drinkingwater/disinfection/nitrification.html. • U.S. Environmental Protection Agency. 2002. Nitrification, Office of Water, USEPA,
Cincinnati, Ohio. • U.S. Environmental Protection Agency. 2001.
National Primary Drinking Water Regulations.
Federal Register, 19(141). • U.S. Environmental Protection Agency. July 2002. List of Contaminants and Their MCLs.
USEPA 816-F-02-013 • U.S. Environmental Protection Agency. 1994.
National primary drinking water regulations; disinfectants and disinfection byproducts; proposed rule. Federal Register, 59, 38668–38829. • U.S. Environmental Protection Agency. 1989.
Drinking Water; National Primary Drinking Water Regulations; Filtration, Disinfection;
Turbidity, Giardia lamblia, Viruses, Legionella, and Heterotrophic Bacteria; Final Rule. Federal
Register, 54(124):27486- 27541.). • Valentine R.L., Ozekin K., Vikesland P.J.
“Chloramine Decomposition in Distribution
System and Model Waters.” AwwaRF, Denver,
Colo., 1998. • Van der Kooij, and D. And Veenendaal, H.R. (2001), Biomass Production Potential of
Materials in Contact with Drinking Water:
Methods and Practical Importance. Water
Science and Technology, 1:3:39. • Verstraete, W., and M. Alexander. 1973.
Heterotrophic Nitrification in Samples of
Natural Ecosystems. Envir. Sci. Technol., 7(39). • Videla, Hector A. (1996), Manual of Biocorrosion,
CRC Press, Boca Raton, Fla. • Walker, J.T., and Morales, M. (1997). Evaluation of chlorine dioxide (ClO2) for the control of biofilms. Water Sci. Technol. 35, 319. • Watson, S.W., F.W. Valos, and J.B. Waterbury, 1981. The Family Nitrobacteraceae. In The
Prokaryotes. Edited by M.P. Starr et al. Berlin:
Springer-Verlag. • Wilczak, Andrzej, Joseph G. Jacangelo,
Joseph P. Marcinko, Lee H. Odell, Gregory J.
Kirmeyer, and Roy L. Wolfe. 1996. Occurrence of nitrification in chloraminated distribution systems. Journal AWWA, 88(7):74-85. • Wilczak, Andrzej, 2001. Chloramine Decay Rate:
Factors and Research Needs. In 2001 AWWA
Annual Conference Proceedings. Washington,
D.C.: AWWA. • Wolfe, Roy L., Nancy I. Lieu, George Izaguirre and Edward G. Means. 1990. Ammonia
Oxidizing Bacteria in a Chloraminated
Distribution System: Seasonal Occurrence,
Distribution, and Disinfection Resistance. Appl.
Environ. Microbiol., 56(2): 451-462. • Wolfe, R.L., and N.I. Lieu. 2001. Nitrifying
Bacteria in Drinking Water. In Encyclopedia of Environmental Microbiology, edited by G.
Bitton. New York: John Wiley and Sons. • Woolschlager, J.E., B.E. Rittmann, P. Piriou and B. Schwartz. 2001. Developing an Effective
Strategy to Control Nitrifier Growth Using the Comprehensive Disinfection and water
Quality Model. In Proc. World Water and
Environmental Resources Congress. Renton,
Vir.: ASCE., Alexandria, Va. S
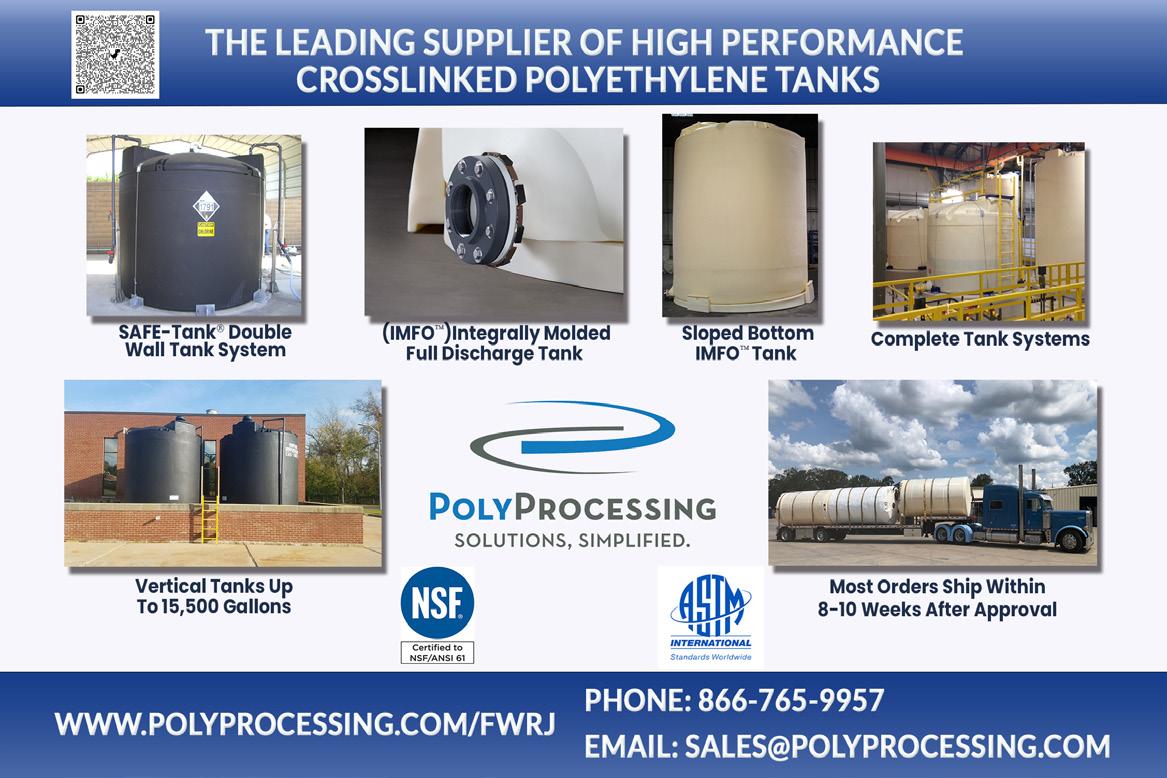